\
GENERAL OVERVIEW OF INFLAMMASOME SENSING AND SIGNALING
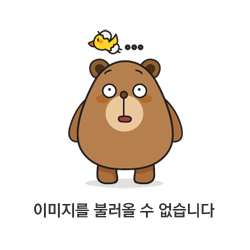
Several cytoplasmic innate immune sensors form inflammasome complexes in response to PAMPs and DAMPs, including AIM2, NAIP, NLRC4, NLRP1, NLRP3, Pyrin, and caspase-11 (Fig. 1); further, mouse NLRP6, NLRP9b, and NLRP12 and human NLRP2, NLRP7, and IFN-inducible protein 16 (IFI16) have also been proposed to activate caspase-1 (5), although the ability of these sensors to form bona fide inflammasome complexes has remained uncertain (29).
Inflammasome sensors initiate a generally proinflammatory signaling cascade upon activation; however, the molecular mechanisms regulating the activation of each sensor and the composition of each inflammasome complex are distinct. Inflammasome sensors differ based on whether they are activated by direct interaction with a ligand or by indirect sensing of broader cellular perturbations (29). AIM2, NAIP, and caspase-11 directly bind to their cognate ligands, whereas NLRP1, NLRP3, and Pyrin respond to cellular perturbations (Fig. 1B).
AIM2 binds exclusively to cytoplasmic double-stranded DNA (dsDNA), independent of the sequence of the dsDNA (30–33).
Similarly, NAIP proteins directly bind flagellin and components of the type III secretion systems (T3SS) of Gram-negative bacteria, such as Salmonella enterica serovar Typhimurium, leading to activation of the NLRC4 inflammasome (discussed below) (34, 35). Mouse caspase-11 and the two human analogs caspase-4 and caspase-5 are all sensors of cytoplasmic lipopolysaccharide (LPS) (36–39) (Fig. 1C).
Direct interaction between the lipid A portion of LPS and the CARD of caspase-11 catalyzes oligomerization of caspase-11 and activation of the noncanonical NLRP3 inflammasome (36, 40–44). While caspase-11 cannot directly cleave pro-IL-1 and pro-IL-18, it can induce pyroptosis in the absence of caspase-1 by cleaving gasdermin D (40). Further, LPS-induced activation of caspase-11 leads to potassium efflux (45) or caspase-11-dependent cleavage of the membrane channel pannexin-1, followed by an ATP release that subsequently activates the purinergic receptor P2X7R and pyroptosis (46).
NLRP3 responds to cellular perturbations emanating from stimulation with PAMPs and DAMPs rather than through binding to an activating ligand (Fig. 1B). These cellular cues include lysosomal disruption and subsequent leakage of cathepsin B (47), potassium efflux via P2X7R (48–51), formation of pores on the cell membrane (52), translocation of cardiolipin from the inner to the outer mitochondrial membrane (53), production of reactive oxygen species (ROS) (54, 55), oxidized mitochondrial DNA (56), calcium influx and a reduction in cellular cyclic AMP (57–59), and alteration of the cell volume (60).
While several molecular mechanisms involving homeostatic disruption have been proposed, a single unifying signal leading to NLRP3 inflammasome activation has yet to emerge. Similar to NLRP3, NLRP1 and Pyrin respond to cellular perturbations rather than to direct binding to a specific ligand.
The mechanism of NLRP1 activation is cleavage dependent; human NLRP1 and mouse NLRP1b are activated by any protein capable of inducing N-terminal proteolytic cleavage of NLRP1 (61–67), demonstrating that these inflammasome sensors are not specific to any single ligand. Activation of Pyrin is more complex. In homeostasis, Pyrin is phosphorylated on two serine residues by the RhoA effector serine-threonine kinases PKN1 and PKN2, promoting interaction between Pyrin and the regulatory proteins 14-3-3 and 14-3-3 (68–70). The interaction between Pyrin and 14-3-3 regulatory proteins holds Pyrin in an inactive state to prevent activation of the Pyrin inflammasome in unstimulated cells (70, 71).
Rho-inactivating toxins and T3SS effectors produced by many bacteria inhibit the action of PKN1 and PKN2, thereby relieving phosphorylation of Pyrin and promoting activation of the Pyrin inflammasome (71–76) (Fig. 1B). The requirement of the inflammasome adaptor protein ASC for the formation of an inflammasome complex also differs among inflammasome sensors (Fig. 1A).
ASC is a bipartite protein comprised of a pyrin domain (PYD) and a CARD. AIM2, NLRP3, and Pyrin carry a PYD but not a CARD and must therefore bind ASC in order to recruit the CARD-bearing procaspase-1. In contrast, NLRP1 and NLRC4 carry a CARD and can therefore directly recruit procaspase-1 in the absence of ASC (77–80). However, optimal secretion of IL-1 is often achieved only when ASC is present in these inflammasome complexes (12, 13, 81), indicating the universal importance of ASC in inflammasome signaling.
Secretion of IL-1 and IL-18 and induction of pyroptosis have instrumental roles in eliciting, magnifying, and perpetuating inflammation and, in most cases, reducing the overall pathogen burden. Both cytokines serve as a bridge between the innate and adaptive immune systems:
IL-1 is a potent promoter of inflammation, immune cell extravasation, and vasodilation with additional capabilities in modulating adaptive immunity (82), while IL-18 triggers local inflammation as well as IFN- γ production in natural killer (NK) cells, CD4 TH1 cells, and CD8 cytotoxic T cells and augments the development of CD4 TH2 cells (83).
In addition to promoting the release of cytokines and DAMPs, another overarching function of pyroptosis is to expel an infected cell from the tissue (84, 85) or to expel pathogens from infected macrophages (86, 87). In contrast, neutrophils release IL-1 without undergoing pyroptosis (88), reflecting the different roles of immune cells in clearing pathogens. Thus, the inflammasome facilitates disruption of the replicative niche exploited by intracellular pathogens and exposes them to potentially less favorable conditions (discussed below).
BACTERIA
A plethora of bacteria engage with and shape the development of the host immune system. Reflecting the coevolution of bacteria and the host immune system, host cells encode innate immune sensors capable of detecting and exploiting the conservation of key bacterial PAMPs (Fig. 2). In this section, we discuss several groups of bacterial PAMPs that are recognized by their respective inflammasome sensors and highlight some of the strategies employed by bacteria to block inflammasome activation.
LPS
The cell wall of bacteria functions to maintain bacterial cell integrity and resist osmotic stress. LPS, a key component of the outer membrane of Gram-negative bacteria, is composed of a lipid A portion, a core oligosaccharide, and an O antigen consisting of repeating glycan subunits. LPSs from many Gram-negative bacteria are recognized by human caspase-4, human caspase-5, or mouse caspase-11 (37–43, 89–91) (Table 1).
Caspase-11 directly binds to the penta- or hexa-acylated lipid A portion of cytoplasmic LPS via its CARD, inducing self-oligomerization and activation (36, 44). Some Gram-negative bacteria, including Porphyromonas gingivalis (92), Yersinia pestis (93), Francisella tularensis (44), and Rhodobacter sphaeroides (36), have altered lipid A structures that cannot activate mouse caspase-11. In contrast, human caspase-4 is able to recognize underacylated LPS from F. tularensis subsp. novicida and Bacteroides vulgatus in macrophages, in addition to detecting penta- or hexa-acylated lipid A (94). This interspecies difference in sensing of distinct LPS moieties may reflect differences in the CARDs of caspase-4 and caspase-11, which share 51% sequence identity (94). Whether caspase-5, which shares only 39% sequence identity with caspase-11 in the CARD (94), can sense a different repertoire of LPS variants or other PAMPs remains to be determined. These inflammatory caspases subsequently trigger activation of the NLRP3 inflammasome upon sensing LPS, giving rise to the nomenclature of the noncanonical NLRP3 inflammasome pathway (Fig. 1C) (40). The entry of LPS into the cytoplasm is an important event that leads to activation of the noncanonical NLRP3 inflammasome. Several mechanisms have been discovered to explain how LPS might reach the cytoplasm of the host cell for sensing by caspase-11. Naturally cytoplasmic pathogens, such as Burkholderia thailandensis and Burkholderia pseudomallei, introduce LPS into the cytoplasm as they escape the pathogen-containing vacuole, thereby driving rapid and robust caspase-11 activation (42). However, vacuole-restricted bacteria, such as Escherichia coli, Citrobacter rodentium, and Vibrio cholerae, can also activate the noncanonical NLRP3 inflammasome (14, 40, 90, 95, 96), implying the existence of alternative routes by which LPS can enter the cytoplasm. In the case of vacuole-restricted bacteria, type I IFN signaling, which is normally induced in response to infection, has an important role in the liberation of LPS in the cytoplasm. Type I IFNs upregulate the expression of host IFN-inducible GTPases, including guanylate-binding proteins (GBPs) and immunity-related GTPases (IRGs) (97). In mouse macrophages, GBPs colocalize with pathogen-containing vacuoles, where they facilitate lysis of the vacuolar membrane such that the bacteria and their associated LPS are released into the cytoplasm for detection by caspase-11 (98, 99). GBPs can also direct IRGB10 to the cell membrane of cytoplasm-exposed Gram-negative bacteria to induce bacteriolysis (100), thereby increasing the amount of free LPS, but also DNA (discussed further below), in the cytoplasm. Bioinformatic analysis of IRG family members identified an amphipathic helix on the C terminus of IRGB10 (100) that is a putative transmembrane region with antimicrobial potential. It is possible that the transmembrane region of IRGB10 may allow insertion into and destabilization of bacterial membranes. In mouse embryonic fibroblasts, pathogen-containing vacuoles carrying Chlamydia trachomatis are decorated with ubiquitin in an IRG-dependent manner (101). This ubiquitination facilitates GBP recruitment to the membrane and subsequent lysis of the pathogen-containing vacuole (101). Therefore, lysis of pathogen-containing vacuoles represents an important mechanism by which LPS from vacuole-restricted bacteria can enter the cytoplasm (102). However, it is noteworthy that in the case of the related bacterium Chlamydia muridarum, recruitment of GBPs to the pathogencontaining vacuole or the bacterial cell wall is not necessary for activation of the inflammasome (103), suggesting a further route by which ligands from this bacterium can be introduced into the cytoplasm. Another mechanism by which LPS can be introduced into the cytoplasm in the absence of cytoplasmic invasion by bacteria is through outer membrane vesicles (OMVs). LPS encapsulated within OMVs, which are shed by Gram-negative bacteria (such as E. coli), can be endocytosed via a clathrin-mediated process (104). This process is followed by escape of the LPS from the endocytic compartment into the cytoplasm of mouse macrophages to induce caspase-11 activation (104). It has also been demonstrated that GBPs encoded on chromosome 3 or GBP2 is involved (105). Mechanistically, isoprenylated GBPs were found to associate with the surface of OMVs or with transfected LPS (106), suggesting that LPS is the PAMP which targets GBPs to bacterial membranes and OMVs. However, the exact mechanism by which GBPs facilitate release of LPS for sensing by caspase-11 remains poorly defined. It is possible that GBPs induce the rupture of OMVs to release LPS into the cytoplasm, perhaps by recruiting additional membrane-disrupting proteins, such as IRGB10 (100). Alternatively, GBPs bound to OMVs may directly facilitate binding of caspase-11 to LPS embedded in the OMV membrane. Sensing of LPS via caspase-11 and activation of the noncanonical NLRP3 inflammasome can lead to a protective or detrimental outcome. For example, injection of LPS into mice induces lethality (18, 40, 41, 44, 107–110). In this case, caspase-11- and gasdermin D-mediated tissue damage and pyroptosis of endothelial cells in response to LPS have been proposed to be responsible for driving the lethality of endotoxemia (18, 40, 44, 95, 111). In contrast, caspase-11 provides host protection in vivo in response to infection by Gram-negative bacterial pathogens, including S. Typhimurium, enteropathogenic E. coli, B. thailandensis, B. pseudomallei, Legionella pneumophila, Acinetobacter baumannii, and Klebsiella pneumoniae (42, 85, 89, 112–114). The increased susceptibility of mice lacking caspase-11 or gasdermin D to these bacteria can likely be attributed to defective activation of pyroptosis and an increased bacterial burden. Indeed, pyroptosis can induce extrusion of infected cells from the epithelium (85). In addition, pyroptosis can release intracellular bacteria into the extracellular milieu to promote phagocytosis and killing by neutrophils (86, 115–117) or can shed infected cells for removal by the host (84, 85). These different experimental systems provide insights into the outcome of caspase-11 activation by Gram-negative bacterial pathogens. In humans, extracellular LPS has also been reported to trigger an alternative NLRP3 inflammasome pathway in monocytes (118). This pathway is propagated by TLR4 – TRIF–RIPK1–FADD– caspase-8 signaling upstream of NLRP3 but differs from both the canonical and noncanonical NLRP3 inflammasomes in that activation of this pathway does not lead to pyroptosis or ASC speck formation and is not dependent on potassium efflux (118). Furthermore, the alternative NLRP3 inflammasome pathway appears to be species and cell type specific, as it is absent in mice and in human macrophages and dendritic cells (118). This TLR4-mediated sensing of extracellular LPS may initiate an early warning system for infection by secreting IL-1 without the cell undergoing pyroptosis. In this way, LPS, one of the strongest bacterium-derived activators of the immune system and inflammasomes, can trigger activation of inflammasome pathways without entering the cytoplasm of human monocytes.
Other Cell Wall Components
There is some evidence that bacterial cell wall components other than LPS, including lipopeptides and muramyl dipeptide, activate the inflammasome (Tables 1 to 3). Both Gram-negative and Gram-positive bacteria have acylated lipopeptides associated with their cell walls, which activate NLRP7 in human macrophages (119). Activation of NLRP7 has been reported to result in ASC-dependent caspase-1 activation and restriction of the replication of Staphylococcus aureus and Listeria monocytogenes (119); however, human macrophages lacking NLRP7 retain some inflammasome activation in response to bacterial infection (119). NLRP3 knockdown also results in decreased IL-1β secretion upon S. aureus and L. monocytogenes infection (119), suggesting that NLRP7 may function redundantly with NLRP3 for inflammasome activation in these infections. Further studies are required to more fully elucidate the relative contributions of NLRP7 and NLRP3 to inflammasome activation in these instances. Cell wall fragments derived from Lactobacillus casei elicit NLRP3 inflammasome activation in mouse vascular endothelial cells (120). Mechanistically, cell wall fragments increase lysosomal permeability and thereby facilitate release of cathepsin B into the cytoplasm to activate NLRP3 (120). The cell surface protein M1 of Streptococcus pyogenes can likewise activate the NLRP3 inflammasome upon clathrin-mediated endocytosis, in a manner dependent on potassium efflux (121). A further study suggests that the muramyl dipeptide of Bacillus anthracis is detected by its cognate cytosolic sensor, NOD2, which has been reported to recruit NLRP1 to mediate caspase-1 activation and secretion of IL-1 (122). However, the mechanistic relationship, if any, between NOD1 and NLRP1 activation remains unclear. Previous studies have shown that NLRP1 is activated by protease-specific cleavage of the N-terminal domain or the function-tofind domain; therefore, NOD2 binding to muramyl dipeptide potentially has some role in triggering this cleavage event. However, it is unlikely that muramyl dipeptide would induce direct cleavage of NLRP1. Taken together, these studies highlight the potential of cell wall components to trigger activation of multiple inflammasomes.
Flagellin and Secretion Systems
Motile bacteria frequently encode specialized organelles, called flagella, which facilitate bacterial movement and attachment. Repeating subunits of the monomeric flagellin protein are the main constituents of bacterial flagella (123). Many Gramnegative bacteria utilize a related but specialized apparatus, called a secretion system, to inject virulence factors into the host cytoplasm. While the virulence factors injected depend on the bacterial species, the secretion system apparatus itself is relatively conserved (124, 125). Many flagellated bacteria trigger activation of the inflammasome via NAIP and NLRC4 (Tables 1 and 2). Cytoplasmic flagellin monomers are initially sensed by murine NAIP5 or NAIP6 (34, 35, 126) and the single human NAIP (127). A stretch of 35 amino acids from the C terminus of flagellin is required to trigger activation of the NLRC4 inflammasome (126). Mutating the inflammasome-activating flagellin residues abolishes motility in L. pneumophila (126), indicating that these residues are critical for flagellin function. Furthermore, structural analysis suggests that upon binding NAIP5, flagellin adopts a conformation similar to its structure in flagellar filaments (128), implying that mutating residues important for NAIP recognition would also disrupt formation of flagellar filaments. Elements that constitute the T3SS apparatus can also be recognized directly by inflammasome sensors (Fig. 2). The T3SS rod proteins of many Gram-negative bacteria are sensed by the murine NAIP2 protein (34, 35, 123, 129) (Table 1); likewise, the T3SS needle proteins of many Gram-negative bacteria are sensed by NAIP1 in mice and by NAIP in humans (35, 130, 131) (Table 1). A further study has indicated that both the inner rod (PscI) and needle (PscF) T3SS proteins of Pseudomonas aeruginosa are sensed by human NAIP in macrophages and peripheral blood mononuclear cells (PBMCs) (132). An additional study broadened the role of human NAIP even further, with evidence that NAIP can recognize the T3SS inner rod proteins of S. Typhimurium, Shigella flexneri, and Burkholderia spp., in addition to flagellin and T3SS needle proteins from these bacteria (133). These studies have therefore shown the single human NAIP protein to be a versatile sensor capable of interacting with multiple PAMPs. Further studies have mapped the nucleotide-binding domain (NBD) of mouse NAIP2, -5, and -6 (134), as well as the LRR domain of NAIP5 (128, 135), as the region responsible for ligand specificity. The evolutionary and functional basis for the different specificities of murine and human NAIPs remains a mystery. Rodent NAIP paralogs are likely to have evolved from expansion or duplication of a single progenitor, with NAIP1, -2, and -3 representing one family and NAIP4, -5, -6, and -7 representing another (136). Furthermore, murine NAIPs have been reported to undergo extensive recombination within regions conferring ligand specificity (134), which may facilitate positive diversifying selection to combat the evolution of bacterial ligands. However, why mice encode several NAIP proteins with specificity for distinct bacterial ligands while humans encode a single NAIP capable of recognizing the same set of ligands remains uncertain. Flagellin must be introduced into the cytoplasm for recognition by cytoplasmic NAIP proteins to occur. Certain bacteria, such as L. monocytogenes, invade the cytoplasm shortly after phagocytosis in order to replicate and thereby directly introduce flagellin and other PAMPs into the cytoplasm (137). However, other bacteria remain in a pathogen-containing vacuole, and their flagellin is therefore sequestered and not exposed to the cytoplasm. In these instances, the T3SS or T4SS apparatus can pierce the pathogen-containing vacuole membrane or host cell membrane to deliver virulence factors and effector proteins, some of which may include flagellin subunits, into the cytoplasm (124, 125). For example, infection with the vacuole-restricted bacteria S. Typhimurium and L. pneumophila triggers activation of the NLRC4 inflammasome, a process which requires a functional T3SS and T4SS, respectively (138, 139). During this process, flagellin subunits can be secreted into the host cytoplasm through these secretion systems (13, 138, 140). Activated NAIPs subsequently bind NLRC4 to initiate inflammasome formation (Fig. 2). Mechanistically, an association between the ligand and NAIP induces NLRC4 binding, causing a conformational change in bound NLRC4 that exposes an oligomerization surface to which additional inactive NLRC4 subunits are recruited to initiate inflammasome formation (128, 141–143). Activation of the NAIP-NLRC4 inflammasome can lead to the recruitment of NLRP3 to the same inflammasome complex (14), which may require phosphorylation of NLRC4 at residue S533 (144–146). A further study has shown that the leucine-rich repeat-containing kinase LRRK2 complexes with NLRC4 in response to S. Typhimurium infection, leading to phosphorylation of NLRC4 at residue S533 and to activation of the NLRC4 inflammasome (147). The ability of NAIP-NLRC4 to recognize flagellin and T3SS apparatus proteins provides host protection against many Gram-negative bacterial infections (13, 14, 148). NLRC4 is highly expressed in mouse enterocytes and intestinal immune cells (149). Studies focusing on S. Typhimurium infection of the intestinal epithelium have suggested the mechanism by which NLRC4 and pyroptosis mediate the host defense against bacterial infection. Specifically, S. Typhimurium-infected wild-type (WT) enterocytes are ejected from the intestinal epithelium via NLRC4-driven pyroptosis (84). This expulsion mechanism is accompanied by the release of eicosanoids and IL-18 and is dependent on NAIP-NLRC4, caspase-1, and caspase-8 (150, 151). Consistent with this observation, caspase-8 is recruited to the NAIP-NLRC4 inflammasome in response to S. Typhimurium infection (152); however, it is robustly activated only with the absence or inhibition of gasdermin D or caspase-1 (19, 153). Phase variation, downregulation, and concealment of ligands are important mechanisms used by bacteria to avoid or minimize detection by the NAIP-NLRC4 inflammasome. For instance, S. Typhimurium switches expression of T3SS proteins from SPI-1 to SPI-2 upon reaching the pathogen-containing vacuole, facilitating the secretion of effector proteins that promote survival in the vacuole; importantly, the SPI-2 rod protein SsaI is not recognized by NAIP (123), further contributing to pathogen survival. Further, a strain of S. Typhimurium that constitutively expresses flagellin is rapidly cleared in mice by the inflammasome pathway (86), suggesting that regulating flagellin expression is critical for bacterial survival. Similarly, L. monocytogenes flagellin expression is suppressed at 37°C (154), potentially allowing evasion of NLRC4 inflammasome detection in the gastrointestinal tract. Interestingly, the flagellins of Campylobacter jejuni and Helicobacter pylori fail to activate the NAIP-NLRC4 inflammasome (145, 155), most likely due to differences in flagellin sequence composition compared to that of flagellins produced by NLRC4-activating bacteria. These flagellins also fail to activate TLR5 (156), allowing these bacteria to evade detection by both known sensors of flagellin. Further, a T3SS-secreted effector protein of pathogenic Yersinia spp., YopK, can bind to its own T3SS translocon (93), thereby masking and preventing recognition of the T3SS by NAIPs. The prevalence of evasion mechanisms directed against the NAIP-NLRC4 inflammasome highlights the importance of this signaling axis in clearing Gram-negative bacterial infections.
Bacterial DNA
Both microbes and mammalian cells use the same basic biomolecules to encode genetic information. Mammalian cells have evolved mechanisms to distinguish self nucleic acids from nonself nucleic acids. DNA of host cells is normally sequestered inside the nucleus and mitochondria; such spatial separation allows cytosolic DNA sensors to respond only to PAMP- or DAMP-associated DNA in the cytoplasmic space (Fig. 2).
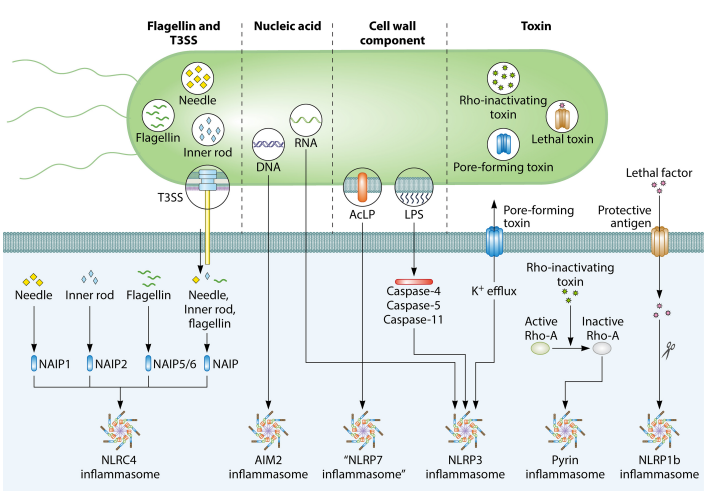
FIG 2 Major bacterial activators of the inflammasome. Four major groups of bacterial components trigger activation of the inflammasome. Flagellin and components of the type III secretion system (T3SS) can be injected into the cytoplasm of the host cell via the T3SS. The T3SS needle and inner rod proteins are sensed by mouse NAIP1 and NAIP2, respectively, whereas flagellin is sensed by mouse NAIP5 or NAIP6. The needle and inner rod proteins and flagellin are all sensed by human NAIP. Ligand-bound NAIPs recruit NLRC4 to the same complex to drive activation of the NLRC4 inflammasome. The bacterial nucleic acid molecules DNA and RNA can activate the AIM2 and NLRP3 inflammasomes, respectively. RNA-DNA hybrids derived from bacteria (not shown) can also activate the NLRP3 inflammasome. The cell wall components acylated lipopeptides (AcLP) might activate a putative NLRP7 inflammasome in human macrophages. Lipopolysaccharides (LPS) from the cell walls of Gram-negative bacteria activate human caspase-4 and caspase-5 and mouse caspase-11. These inflammatory caspases oligomerize and drive activation of the NLRP3 inflammasome. Pore-forming toxins produced by bacteria induce K efflux, a physiological aberration sensed by the NLRP3 inflammasome. Rho-inactivating toxins inactivate the host GTPase RhoA, which relieves inhibition of Pyrin, leading to activation of the Pyrin inflammasome. The protective antigen of lethal toxin generates pores on the host cell membrane, allowing lethal factor to enter the cytoplasm and mediate cleavage of NLRP1b. Cleaved NLRP1b induces formation of the NLRP1b inflammasome.
The inflammasome-forming DNA sensor AIM2 responds to infections by a range of Gram-negative, Gram-positive, and Gram-variable bacteria (Tables 1 to 3).
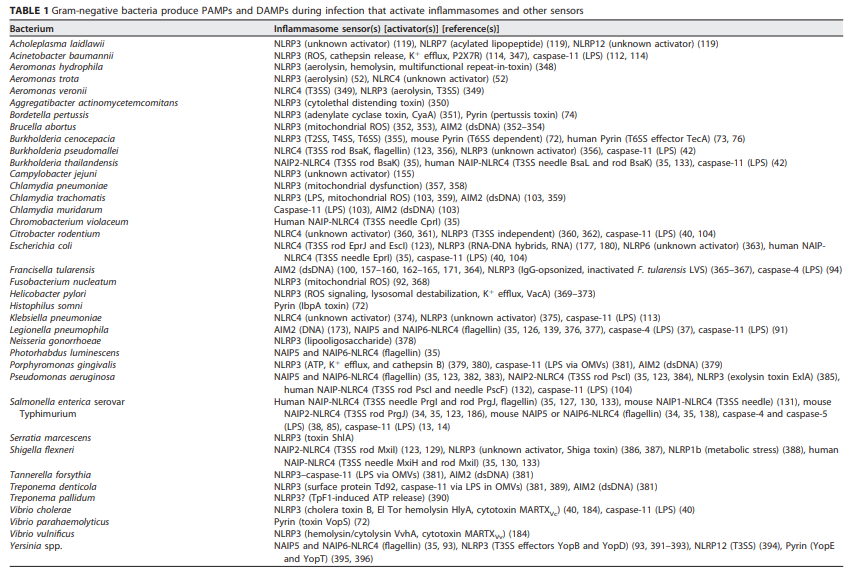
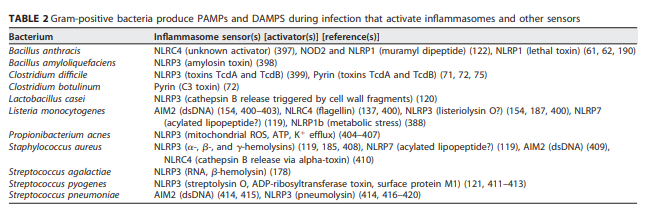
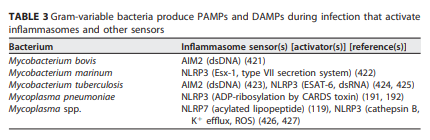
The mechanism by which DNA, normally sequestered within the cell membrane and cell wall of bacteria, becomes exposed in the cytoplasm for inflammasome sensing during infection of a host cell has begun to be unraveled by use of the intracellular Gram-negative bacterium Francisella novicida as a model. Upon infection of the host cell, F. novicida is contained within a pathogen-containing vacuole; however, in order to replicate, F. novicida escapes from the pathogen-containing vacuole via a T6SS and invades the cytoplasm (157).
Initial studies found that mutant strains of F. novicida that are unable to escape the pathogen-containing vacuole fail to activate the AIM2 inflammasome in macrophages and dendritic cells (158, 159). Furthermore, mutant strains of F. novicida that are prone to intracellular lysis exhibit an increased ability to trigger activation of the AIM2 inflammasome in macrophages (160).
This finding suggests that bacteriolysis is an important mechanism in the liberation of bacterial DNA for AIM2 sensing. Further studies have provided insights into the mechanisms by which F. novicida is lysed during infection. Type I IFN signaling is induced during infection with F. novicida and is required for inflammasome activation (161). This signaling cascade leads to the upregulation of IFN-inducible proteins, including GBPs and IRGs, by the transcription factor IRF1 (162).
Evidence indicates that GBP members GBP2 and GBP5 target cytoplasmic F. novicida and recruit the IRG member IRGB10 to the bacterial cell membrane (100), where they instigate bacteriolysis to induce the release of bacterial DNA into the host cytoplasm for sensing by the AIM2 inflammasome (100, 162, 163).
Consistent with the role of the AIM2 inflammasome in antibacterial host defense, mice lacking AIM2, GBPs, or IRGB10 are highly susceptible to infection by F. novicida and have an impaired ability to induce activation of the inflammasome (158, 162–167). Cytoplasmic DNA sensing in certain human myeloid cells has been proposed to employ an alternative pathway that is independent of AIM2 (168). Upon F. novicida infection, cGAS and STING induce lysosomal cell death, potassium efflux, and subsequent activation of the NLRP3 inflammasome in an AIM2-independent manner (168).
However, why AIM2 is not functional in human monocytes was not addressed in that study. Indeed, AIM2 is the major DNA-sensing inflammasome pathway in THP-1 cells, monocyte-derived dendritic cells, and keratinocytes in humans and in myeloid cells in mice (169, 170), suggesting that this alternative DNA-sensing inflammasome pathway is cell type rather than species specific. Further, this alternative pathway may have some capability to compensate the AIM2 inflammasome pathway in human monocytes when it is inhibited by certain virulence factors. Several bacteria strategically prevent DNA release and activation of the AIM2 inflammasome to ensure survival and dissemination in the infected host. F. novicida encodes a lipid II flippase, MviN, and a CRISPR-Cas system to enhance the integrity of the bacterial envelope and to resist bacteriolysis and subsequent release of DNA (171, 172). Similarly, L. pneumophila produces an effector protein, SdhA, that helps to maintain membrane integrity of the pathogen-containing vacuole (173). In the absence of SdhA, more L. pneumophila DNA is released into the host cytoplasm, activating the AIM2 inflammasome and pyroptosis within 5 h of infection of human macrophages (173). It is likely that other cytosolic bacteria have similar strategies to limit the amount of free bacterial DNA in the cytoplasm. For example, S. flexneri encodes an E3 ubiquitin ligase, IpaH9.8, which mediates ubiquitination and degradation of mouse and human GBPs (174–176). Given the importance of GBPs in mediating bacteriolysis, IpaH9.8 potentially prevents GBP-induced binding to bacteria and subsequent bacteriolysis and DNA release. Additional searches for similar cytosolic bacterial virulence factors that promote degradation of GBPs and IRGs or DNA sensors would further unveil the complexity of the microbial DNA sensing pathway.
Bacterial RNA and Other Bacterial Nucleic Acids
Bacterial RNA species activate multiple cytoplasmic RNA sensors, including the inflammasome sensor NLRP3 and the noninflammasome RLRs and non-RLR helicases. Purified total RNAs derived from both Gram-positive and Gram-negative bacteria trigger activation of the NLRP3 inflammasome in mouse macrophages (177, 178). In addition, both mRNA and RNA-DNA hybrids of E. coli activate NLRP3 in mouse macrophages and dendritic cells (179, 180). Further studies indicate that bacterial mRNA, tRNA, and rRNA are all capable of activating NLRP3 in human macrophages (181). It has been proposed that bacterial RNA might serve as an important “vita-PAMP” to signify the presence of viable microbes to host sensors (179).
mRNA from bacteria, but not that from eukaryotic cells, activates the NLRP3 inflammasome, and 3= polyadenylation of bacterial mRNA abolishes its inflammasome-activating activity (179), suggesting that the 3= end of bacterial mRNA might be important for NLRP3 engagement and that polyadenylation of host RNA might be sufficient to suppress immune recognition of self RNA. However, the mechanism by which NLRP3 senses and is activated by bacterial RNA remains poorly defined. Two hypotheses have been postulated to describe how bacterial RNA species might activate NLRP3. First, bacterial RNA and RNA-DNA hybrids colocalize with NLRP3 and caspase-1 inflammasome specks in mouse macrophages infected with E. coli (180), providing some evidence that NLRP3 may interact with bacterial RNA.
However, whether this finding reflects a direct interaction between NLRP3 and bacterial RNA or the requirement of an RNA-binding mediator has yet to be shown conclusively. Second, the RNA helicase DHX33 may function as an upstream sensor of bacterial mRNA and subsequently interact with and activate NLRP3 (182, 183). Further studies are therefore required to elucidate whether cytoplasmic bacterial RNA activates NLRP3 directly, through an additional sensor, such as DHX33, or via a mechanism involving nonspecific homeostatic disruption. It would also be interesting to investigate how bacterial RNA molecules gain access to the cytoplasm to more fully elucidate the molecular mechanisms governing the host response to bacterial RNA.
Toxins
Bacteria encode an arsenal of toxins that modify the function, metabolism, and physiology of the host cell to favor bacterial infection. However, the host immune system can sense some bacterial toxins and activate the inflammasome in response to bacterial infection. Inflammasome activation by bacterial toxins is generally achieved through the pore-forming and/or enzymatic activity of the toxin (Fig. 2).
Many bacterial toxins function by forming pores in the host cell membrane. Certain pore-forming toxins produced by both Gram-positive and Gram-negative bacteria are indirectly sensed by the NLRP3 inflammasome (Tables 1 and 2). Mechanistically, toxininduced pore formation in the host cell membrane can cause potassium efflux (51), which in turn activates the NLRP3 inflammasome (Fig. 2). Indeed, potassium efflux has been proposed as a common upstream activator of the NLRP3 inflammasome in response to bacterial toxins (51).
However, the presence of other bacterial PAMPs in concert with potassium efflux appears to be important for NLRP3 inflammasome activation by some pore-forming toxins. For instance, the Gram-negative pathogenic bacteria V. cholerae and Vibrio vulnificus (184) and the Gram-positive bacterium S. aureus (185) produce hemolysin toxins that induce potassium efflux in macrophages. Culture supernatants derived from V. cholerae can trigger potassium efflux and activation of caspase-1 via the NLRP3 inflammasome in macrophages; however, purified hemolysin induces substantially less caspase-1 activation (184).
It is possible that other bacterial PAMPs, such as peptidoglycan, may provide a priming signal (signal 1) to upregulate inflammasome components, while toxin-induced potassium efflux acts as the inflammasome-activating signal (signal 2). In this way, hemolysins may function in concert with other bacterial virulence factors to maximally activate the NLRP3 inflammasome. Introducing pores into the pathogen-containing vacuole membrane can also permit bacterial escape, and subsequent release of PAMPs, into the cytoplasm for innate immune sensing. For instance, the L. monocytogenes toxin listeriolysin O is required for bacterial invasion of the cytoplasm from the pathogen-containing vacuole (186). A mutant strain of L. monocytogenes lacking listeriolysin O fails to induce IL-1 secretion in macrophages (186), likely due to inflammasome-activating PAMPs remaining sequestered in the pathogen-containing vacuole. A further study implicated listeriolysin O-mediated rupture of phagosomes and release of cathepsin B as the main mechanism by which NLRP3 is activated upon L. monocytogenes infection of human PBMCs (187).
Similarly, Streptococcus agalactiae produces a -hemolysin toxin which forms pores in the pathogen-containing vacuole and presumably releases RNA and lysosomal components into the cytoplasm for sensing by the NLRP3 inflammasome (178). The lethal toxin produced by B. anthracis performs both pore-forming and enzymatic activities that lead to activation of the NLRP1 inflammasome. The lethal toxin is a two-component toxin comprising a pore-forming protective antigen and an enzymatically active lethal factor (188). The protective antigen facilitates lethal factor entry into the host cell cytoplasm, where the lethal factor cleaves mouse NLRP1b in the N-terminal region, leading to formation and activation of the NLRP1b inflammasome (61–64, 66). Mice carrying a cleavage-resistant variant of NLRP1b are unable to sense B. anthracis infection and succumb to infection faster than WT mice do (189, 190). Proteolytic cleavage therefore represents one mechanism by which the inflammasome can be activated by bacterial toxins. Posttranslational modifications induced by enzymatically active toxins represent an additional mechanism by which the inflammasome can be activated. For instance, Mycoplasma pneumoniae encodes an ADP-ribosylating and vacuolating toxin, designated community-acquired respiratory distress syndrome (CARDS) toxin (191), which activates the NLRP3 inflammasome by direct ADP-ribosylation of NLRP3 (191). Mice lacking NLRP3 and infected with M. pneumoniae are unable to produce IL-1 and exhibit delayed bacterial clearance during acute pulmonary infection (192), clearly indicating a role for the NLRP3 inflammasome in bacterial clearance; however, whether activation of the NLRP3 inflammasome by the CARDS toxin directly relates to the observed in vivo protection from M. pneumoniae infection remains unclear. It would therefore be prudent to generate a mutant M. pneumoniae strain lacking the CARDS toxin to more clearly define the contribution of this toxin to bacterial clearance in vivo.
Posttranslational modification and inactivation of Rho family GTPases by enzymatically active bacterial toxins can also indirectly activate the Pyrin inflammasome. Pyrin is normally phosphorylated by host RhoA serine-threonine kinases PKN1 and PKN2, promoting Pyrin inhibition by 14-3-3 proteins (68–70). Bacterial toxins posttranslationally modify Rho family GTPases in the I-switch region and inhibit RhoA-dependent kinase activity, thereby relieving inhibition of Pyrin and licensing activation of the Pyrin inflammasome (68, 71, 72). Specifically, the Clostridium difficile cytotoxins TcdA and TcdB monoglucosylate RhoA, the Clostridium botulinum toxin C3 ADP-ribosylates RhoA, and the Vibrio parahaemolyticus toxin VopS and the Histophilus somni toxin IbpA adenylate RhoA (71, 72, 74, 75). These studies highlight that diverse posttranslational modifications induced by bacterial toxins can be sensed by multiple inflammasome sensors.