1. Introduction
There is an increasing worldwide demand for seafood due to the awareness of fish as an important protein source for a growing population. Wild fisheries are currently in a state of decline because of overfishing, climate change, pollution and marine habitat destruction, among other factors. However, aquaculture is rising all around the world. Du to commercial and production reasons, farmed fish are held in much more crowded conditions than those existing in the wild, with the consequence that farmed fish are more stressed and hypersensitive than savage ones. Previous studies agreed that the response of fish to stress conditions depends on the stressor (temperature, crowding, hypoxia, the presence of heavy metals, etc.), and also on the characteristics of the fish themselves (e.g. fish species, age or sex) [1,2].
Such stressful conditions could enhance the spread of pathogenic bacteria and cause serious outbreaks of disease. Indeed, the diseases caused by bacterial infections and the low survival rate of the fish represent significant challenges to fish farmers [3]. Given that bacteria can survive well in aquatic environment independently of their hosts, bacterial diseases have become major impediments to aquaculture [4]. Both prophylactic and therapeutic treatments use drug supplemented feeds to keep farmed fish free of diseases [5]. Unfortunately, the indiscriminate use of antimicrobial agents has led to the development of resistant strains and the search for alternatives to antibiotics [6].
Diseases caused by antibiotic-resistant bacteria are difficult to treat, and there are only a few new antimicrobial compounds in the drug development pipeline. Likewise, antibiotics may reduce larval growth and inhibit the defence mechanisms of the fish larvae [7]. Besides sensitization reactions and other undesirable side effects on fish, it has been demonstrated that between 60% and 73% of antibiotics and related chemical drugs administered to farmed fish are excreted with the faeces [8].
In our point of view, a strategy that combines the pathogen, the host and the environment characteristics will be the most effective method in the longterm to improve aquatic animal health. In the aquaculture industry, when fish diseases are diagnosed, the treatments starts with a global identification of the pathogen and, subsequently, with the administration of antibiotics in water or mixed with feed pellets in an attempt to eradicate the pathogen. Unfortunately, these administration methods usually neglect the adhesive capacity of bacteria and their ability to arrange themselves as a biofilm [9].
New studies on fish pathogenic bacteria are required in order to understand:
(i) their adhesion mechanisms and factors influencing the same in aquatic environment, (ii) the interaction mechanism established between pathogen and host cells and (iii) the factors involved in virulence, colonization and fish disease.
The adhesion of bacteria to mucus seems to be a prerequisite for successful infection, so that more works focusing on adhesins (which can be considered primary virulence factors), exopolymers and flagella, which play an important role in establishing the initial interaction with mucosal surfaces or cells, are needed to understand the initial steps of mucosal infection as well as those involved in bacterial virulence.
Concomitantly, new studies attempt to better understand the physico-chemical properties of skin mucus and immune components (constitutive or induced upon infection), this will help in the design of appropriate preventive strategies in order to offer better protection in fish against invading pathogens.
Furthermore, more studies are needed to understand the interaction of pathogen and fish cells under different environmental conditions (temperature, salinity). Complicated local signaling arrangements are present in the fish skin but intracellular signaling pathways induced by pathogen are still less studied. Further studies are necessary to help us understand these immune reactions in order to develop new practices that will improve the health and welfare of farmed fish [10].
To the best of our knowledge, this is the first review focuses on the interaction between bacteria and fish cells, with emphasis on the importance of the link between adhesion capacity, interactions occurring in biofilm, the parameters influencing bacterial virulence, the role of biofilm in pathogenicity and the susceptibility of pathogenic bacteria to antibiotics.
The well understanding of the link between all these parameters could prevent important fish diseases that cause serious economic lost in aquaculture.
2. Adhesion of pathogenic bacteria and biofilm formation
The survival, multiplication and pathogenicity of bacteria require the existence of both pathogen and fish as well as the successful invasion of the host fish by the pathogen. It was reported long ago that adhesion is a prerequisite for successful infection.
A deep study of the mechanisms implicated in the adhesion mechanism could intensely contribute on protecting fish from diseases. Although the number of fish pathogens identified is relatively limited compared to other pathogens, most studies have focused on treatment strategies essentially, by antibiotics. However, information is lacking concerning the adhesion of pathogens to the skin and gut mucus of fish.
2.1. Adhesion of pathogenic bacteria
Adhesion is an important factor in bacterial pathogenicity because it promotes the delivery of bacterial toxins and precedes penetration into target cells by the microorganism. Specific adherence is mediated through compounds on the surface of the bacteria, which bind via rigid stereo-chemical bonds to particular molecules on the support to which it is adhering [11].
Any study of pathogen bacteria adhesion to the host fish should starts with a clear understanding of the mechanisms and the factors involved in the attachment of bacteria to the fish. The initial attachment of bacterial cells to a surface (e.g. mucus) involves many factors, including hydrophobicity, surface charge, surface roughness, surface micro-topography and water flow, components of the surface, pH of the milieu and viscosity, among others [12].
The adhesion process of bacteria to surface and fish mucus, the different forces involved, the successive steps and the specific adhesion receptors that facilitate strong adhesion was discussed in detail in our previous review [10]. In the present review, we focus on the adhesion mechanism in relation with pathogenicity and as a factor that might influence virulence.
For the pathogenic bacteria, it seems that a natural selection process is operating, whereby the most adhesive and resistant bacteria survive to induce disease. Many studies have indicated that external fish mucus has a protective role and evolved robust mechanisms that can trap and immobilize pathogens before they can contact epithelial surfaces. Indeed, the mucus layer is impermeable to most bacteria and many pathogens (reviewed by Refs. [13] and [10]).
However, other studies have suggested that fish mucus could act as a nutrient source for the pathogenic bacteria, and that the microorganisms could persist within the aquatic environments, using mucus of reservoir fish hosts similar to other fish pathogens [11,14]. Regardless of whether the mucus in itself serves as a nutrient or whether it serves as a substance to collect nutrients, it represents a microenvironment that harbors pathogenic bacteria. Being near to each other, these bacteria will multiply and aggregates. Although the exact aggregation capacity, aggregation kinetic and adhesion kinetic will depend on the bacteria, the mucus represents a substratum that provides cohesive forces [15].
Biochemical interactions between the bacteria and mucus, cells or tissues may be enabled by a hydrated matrix of exopolymers, capsular material including exopolysaccharides (EPSs) and others that provides a buffer against sudden changes in the adjacent osmotic environment [16,17]. Such a stable environment may aid in the localization of secreted exoenzymes, which are essential for the adhesion mechanism and the sequestration of nutrients [17,18]. Some bacterial surface structures such as, adhesin, flagella, pili and fimbriae, extend from the bacterial surface to mediate attachment, while attachment receptors bind to specific ligands on the surface. Bacterial adhesin mediates binding to the epithelial extracellular matrix by recognizing specific carbohydrate structures [19].
Bacterial exoproducts, such as outer membrane protein or extracellular polysaccharides, may be involved in adhesion, and there is also evidence supporting the involvement of lipopolysaccharides [20,21]. These polysaccharides may mediate carbohydrate-carbohydrate interactions that are important for adhesion. Once adhesion occurs, the bacteria can induce the expression of other virulence genes and cause the activation of host cell signaling pathways [10,22,23]. Thus, organisms may undergo specific molecular changes to become pathogenic or establish biofilms. Furthermore, several studies have reported that the flagellum is involved in directing the adhesion mechanism. For example, Pseudomonas strain was observed to attach to hydrophilic surfaces via flagella, while assuming random orientations on hydrophobic surfaces [24,25].
Experiments with the pathogens P. aeruginosa [25] and Aeromonas hydrophila [26] demonstrated the important role of flagella in establishing the initial interaction with mucosal surfaces or cells. It was reported that flagellar rotation increases the attachment rates of Escherichia coli bacteria to glass [27], although it could not be ascertained whether the bacteria were adhering by their cell bodies or by their flagella. Morisaki et al. [28] studied the attachment of Vibrio alginolyticus, Vibrio alcaligenes and Alteromonas spp., and concluded that the attachment of these bacteria depended on the electrostatic energy interaction between the polymers existing on the bacterial cell surface and the polymers that exist on the attachment surface. Hence, the role of high ionic conditions such as sea water in the bacterial attachment process was confirmed.
2.2. Mechanism of biofilm formation
After achieving the adhesion stage, the bacteria transform from planktonic to sessile mode of growth. The pathogenic bacteria start their proliferation, aggregate and invade the surface to form a biofilm. Although the biofilm formation is a highly complex process, but it could be abridged in the formation of micro-colony followed by a three dimensional structure formation, biofilm formation and finish by maturation and detachment (dispersal) [29] The infectious bacterial biofilms grow by proliferation and production of an extracellular matrix that consists of a multitude of components of different chemical nature, including exopolysaccharides, proteins, DNA, and other polymers. The function of the matrix is to provide adhesion between bacterial cells, thereby enabling the formation of a multilayered biofilm [30]. In their study focusing on the spatiotemporal dynamics during the establishment of microcolonies, Duvernoy et al. [31] found that adhesion forces of the rod-shaped bacteria E. coli and Pseudomonas aeruginosa are polar. The higher asymmetry generates more circular microcolonies but decreasing either adhesion forces or the asymmetry in their distribution generates more elongated microcolonies. McLay et al. [32] reported that the changes in the average propensity of bacteria to adhere to interfaces are correlated with the average degree of fimbriation. The detachment of planktonic bacterial cells from the biofilm occurs due to mechanical stress or as a result of programmed detachment process. Effectively, Dispersing of biofilm cells occur either by detachment of new formed cells from growing cells or dispersion of biofilm aggregates due to flowing effects or due to quorum-sensing. Although the bacterial detachment is followed by a cessation of EPS production, Dispersed cells from the biofilm have the ability to retain certain properties of biofilm, such as antibiotic in-sensitivity. The cells which are dispersed form biofilm as result of growth may return quickly to their normal planktonic phenotype [29].
In the biofilm, the organized communities of aggregated cells embedded in a hydrated matrix of extracellular polymeric substances showed coordinated behaviour, with the formation of complex threedimensional structures and functionally heterogeneous bacterial communities. Populations of bacteria within biofilms exhibit differences in the expression of surface molecules, antibiotic resistance, and nutrient utilization and virulence factors [33]. Bacteria in biofilms also coordinate their behaviour through cell–- cell communication using secreted chemical signals. Cell signaling allows bacteria to sense and phenotypically respond to their environment, for example, by assessing cell density (also called quorum sensing or QS) or environmental cues [34–36]. Couvigny et al. [37] studied some genetic factors of S. salivarius that dictate host adhesion and biofilm formation and found that in adhesion and auto-aggregation process occur twelve mutations affecting this auto-aggregation phenotype.
The mutations targeted genes encoding (i) extracellular components, including the CshA surface-exposed protein, the extracellular BglB glucan-binding protein, the GtfE, GtfG and GtfH glycosyltransferases and enzymes responsible for synthesis of cell wall polysaccharides (CwpB, CwpK), (ii) proteins responsible for the extracellular localization of proteins, such as structural components of the accessory SecA2Y2 system (Asp1, Asp2, SecA2) and the SrtA sortase, and (iii) the LiaR transcriptional response regulator [37].
The production and the control of bacterial biofilm in Vibrio fischeri depends on a complex regulatory network that controls transcription of the symbiosis polysaccharide (syp) gene locus. In addition to this transcriptional control, biofilm formation is regulated by two proteins, SypA and SypE, which may function in an unusual regulatory mechanism known as partner switching [38].
Gonzalez-Contreras et al. [11] demonstrated that 15 isolates of Streptococcus phocae strains isolated from diseased Atlantic salmon exhibited a similar ability to attach to the Chinook salmon embryo (CHSE) cells line. However, the invasion results showed that the 15 S. phocae isolates tested were not able to enter CHSE cells, showing invasion values of 0%–0.42% of the initial CFU numbers. The same results were obtained with V. anguillarum ATCC 43307. This study indicates that S. phocae can overcome the killing capacity of mucus and serum and proliferate in them. These results could be associated with the presence of a capsular layer around the cells [11]. It is known that many virulence genes of pathogenic bacteria are transcribed more efficiently at higher growth temperatures. In this regard, it was reported that cascades of virulence factors are induced following the P-pili mediated binding of E. coli to its host cell receptor [39]. Studies on Vibrio cholerae, Aeromonas species, and Shewanella oneidens suggested that flagella and mannose-sensitive hemagglutinin (MSHA) pili contribute to biofilm formation. Moreover, mutations in each minor or major pilin gene significantly reduced the bacterium's ability to adhere and form biofilms [40].
Pili are also involved in several other bacterial processes, including bacterial auto-aggregation, target tissue specificity and natural competence for DNA uptake [41]. A. salmonicida subsp. salmonicida, a fish pathogen bacterium, contains a complete set of genes in its genome for two type IV pilus systems, Tap and Flp. Tap-pilus contributes moderately to virulence [42]. However, Bordas et al. [14] reported that the adhesive capability for skin mucus does not seem to be an essential virulence factor of pathogenic strains of Vibrio, since this specific interaction depended on several environmental factors, temperature and, most importantly, salinity. Other studies revealed that the initial attachment and adhesion of pathogen depended on the bacterial surface saturation kinetic. In fact, polar flagellated bacteria did not exhibit surface saturation kinetics, with a few exceptions noted for V. anguillarum and V. cholerae. It was suggested that the adsorption kinetics and the adsorption mechanisms of polar flagellated bacteria were different from those of laterally flagellated microorganisms, which always showed surface saturation kinetics [43].
The involvement of flagella in biofilm formation is not limited to its initiation. Flagellum-mediated motility was suggested to be required for biofilm growth through recruitment of motile cells from the planktonic phase [44]. A difference in pathogen bacterial aggregation was also noted between polarly and laterally flagelled bacteria, this difference in behaviour between pathogens (e.g. V. alginolyticus strains) could be due to the higher formation of extracellular material by the same strains. This material could mask other superficial components which could be involved in adherence, preventing the binding with different sites on the surface of the mucus [14]. In B. subtilis, a high number of regulators acting at the transcriptional level were found to control biofilm formation including DegU/ DegS, Spo0A, SinI/SinR, SlrR/SlrA and AbrB. The resulting action of these regulators is a switch between motility and exopolysaccharide matrix production, and the B. subtilis Pellicle contains subpopulations of motile and of exopolysaccharide-producing cells [44].
3. Parameters influencing pathogenicity
Although virulence in several pathogenic bacteria is governed by chromosomal genes, many studies have claimed that virulence intensity is not stable and that many parameters could influence the magnitude and the expression of virulence factors. To overcome stressful conditions, some pathogens modify the cell membranes to enhance the ability to colonize, localize specific tissues, and avoid the host immune defences [45].
Other bacterial species enter a physiologically viable but non-culturable (VBNC) state as long as they are under chemical and/or environmental stress. Bacteria coordinately regulate these survival mechanisms in environmental conditions, where they confer a selective or pathogenic advantage [46]. Thus, in fish pathology, we suggest that the eradication of bacterial diseases will be obvious if we first increase our knowledge about the factors that influence virulence mechanism. Related to the cell structure or extracellular products (ECPs), virulence mechanisms depend on environmental parameters (i.e., temperature, osmolality, pH, and concentrations of specific ions, salinity) [46,47].
The availability of iron has been shown to be a critical factor in the pathogenicity of microorganisms invading living hosts. For example, Magariños et al. [48] confirmed the importance of iron in the pathogenesis of Pfiesteria piscicida toward fish and showed that the iron sequestering system differs, at least in some aspects, from those reported for other Pasteurella spp. Rasmussen-Ivey et al. [49] suggested that outbreaks of A. hydrophila are generally linked with changes in host susceptibility caused by environmental changes, such as hypoxic conditions and excessive nitrite levels in farmed fish, as well as increases in temperature, which are linked with the production of virulence factors, such as cytotoxins and haemolysins [50,51]. Temperature change has also been demonstrated to induce bacterial membrane remodelling and is required for the maintenance of optimal membrane architecture [52,53]. In addition to change of the pathogenic bacteria from culturable state to VBNC state and temperature, several other factors such as antibiotic pressure, starvation, chlorination and change in the pH have been reported to influences pathogenicity.
3.1. Bacterial modulation to viable but non-culturable (VBNC) state
The bacterial infection mode is considered as an important parameter that influences bacterial pathogenicity. Several studies have demonstrated that aquatic pathogens such as Tenacibaculum maritimum, Flavobacterium psychrophilum, Vibrio sp., A. salmonicida, and Aeromonas hydrophila [54–58] are capable of entering a viable but non-culturable (VBNC) state, and although some of them are capable of recovering their virulent properties, most of them survive in a non-infective state that require a complicated process to restore their virulence capacity. In the VBNC state, the bacteria lose the ability to grow in bacteriological media but maintain viability and pathogenicity and sometimes are able to revert to regular division upon restoration of normal growth conditions [59].
The physiological consequence of VBNC/metabolically active but non-culturable (ABNC) state could be an adaptive effect that supports long-term survival under unfavorable conditions or an after effect of cellular deterioration, which conserves specific features of viable cells but results in a loss of culturability with available techniques [60]. In recent years, gene expression and the protein modulation of VBNC cells have been demonstrated. In fact, for V. parahaemolyticus under VBNC state, several up-regulated proteins associated with transcription (homologs of alpha subunit DNA-directed RNA polymerase and phosphoribosylaminoimidazole carboxamide formyltransferase/ IMP cyclohydrolase), translation (ribosomal protein S1, homologs of elongation factor TU, and elongation factor EF-G), ATP synthase (F1 alpha subunit), gluconeogenesis-related metabolism (dihydrolipoamide acetyltransferase and glyceraldehyde 3-phosphate dehydrogenase), and antioxidants (homologs of peroxiredoxins, AhpC/Tsa family) [60].
Along with simple reversal of the inducing stresses, a variety of interesting chemical and biological factors have been shown to allow resuscitation, including extracellular resuscitation-promoting proteins and a novel quorum-sensing system (AI-3). A group of extracellular bacterial proteins, known as ‘resuscitation-promoting factors’ (Rpfs), co-culture with several eukaryotic cell lines or higher organisms (e.g. protozoans) were able to reactivate dormant cells [57]. Favorable growth conditions with a source of energy and an ideal stoichiometric ratio of carbon to inorganic elements can reverse the VBNC state. Sodium pyruvate restores the biosynthesis of macromolecules such as DNA and proteins, thereby converting the VBNC cells to a culturable state [60]. Likwie, it was reported that, the addition of Tween 20 (3%) or catalase (1%) to a culture of S. Enterica serovar Typhi allowed the VBNC coccoid cells to become culturable again within 24–48 h [61].
3.2. Temperature
The importance of the temperature in the adhesion of fish pathogenic bacteria has been demonstrated by Laurencin and Germon [62] in experiments carried out with V. anguillarum infecting rainbow trout (Oncorhynchus mykiss Walbaum, formerly Salmo gairdneri Richardson). The minimal values of adhesion for all strains were observed at the lowest values of temperature (48 °C) and salinity (10‰) tested. In their study Chen et al. [63] reported that the temperature and proteolytic enzymes influence bacterial adhesion because they can change the mucus composition. Similarly, changes in water temperature cause outbreaks of many fish bacterial diseases in fish farms. Outbreaks occur when water temperature drops to a certain value: Cold water vibriosis, [64]; Cold water disease [65]; Redmouth disease [66]. By contrast, outbreaks of Lactococcosis [67], Haemorrhagic septicaemia [68] and Edwardsiellosis [69] are related to an increase in water temperature [70]. Aeromonas hydrophila is an opportunistic pathogenic bacteria that could infect animals including fish and human. In the non-culturable state, A. hydrophila lost its ability to lyse human erythrocytes and adhere McCoy cells. After temperature-induced resuscitation (from 5 to 23 °C), however, it regained its virulence properties. V. cholera has transformed into coccoid cells in an aquatic microcosm after incubation for 60 days at 4 °C [60,71]. Y. pestis became non-culturable by normal laboratory methods after 21 days in a low-temperature tap water microcosm. We further show evidence that, after the loss of culturability, the cells remained viable by using a variety of criteria, including cellular membrane integrity, uptake and incorporation of radiolabeled amino acids, and protection of genomic DNA from DNase I digestion [72]. It is important also to remember that Gram-negative bacteria can synthesize modified forms of lipid A in response to environmental stimuli and temperature change [73,74].
3.3. Nutrient availability and other environmental stress factors
In aquatic environment nutrient deprivation can be a major selection pressure for bacteria, causing changes in bacterial cell structure and life cycle strategies [59]. This selection pressures in the environment may drive virulence evolution. E. faecalis cell morphology developed a rippled cell surface with irregular shapes when it was cultured in a nutrient-poor microcosm, but after 85 days, 10–30% of the cells were still culturable [75]. The fish pathogen Flavobacterium columnare strains kept under starvation were reported to suffer from structural changes that recovered after culture in standard media, and the virulence of these bacteria after the two weeks ’Starvation decreased, along with their growth rate. Changing morphotype in F. columnare has trade-offs in making bacteria less virulent and thus unable to invade and exploit the host [76]. Enger et al. [77] reported that a problem encountered during detection and enumeration of cells of starving bacteria by the use of immunofluorescence techniques, is the possibility of changes in antigenicity during starvation. Such changes in expression of surface antigens may also reflect changes in infectivity or virulence of the cells. The results obtained by Soto and Revan [47] indicate that culturable Francisella noatunensis subsp. orientalis persist for longer periods of time and in a higher numbers in seawater and its persistence is inversely related to water temperature. They reported also that pathogenic properties of F. noatunensis subsp. orientalis suspended in water microcosms appear to decrease after only 24 h and become non-infective after 2 days in the absence of the fish host. Romalde et al. [78] verified that Y. ruckeri -stressed or nutrient-starved cells may enter a state of dormancy, remaining infective but unable to grow in normal laboratory media. Likewise, for Y. ruckeri, isolated from a french fish farm, proteomic analysis revealed differences in the outer membrane protein (OMP) pattern between immobilised and planktonic cells affecting proteins involved in motility, transport, metabolism, adaptation, etc. [79]. It is important to consider that the differences between these two physiological states cannot be extrapolated to other more complex situations during the infection process. On the other hand, the results of adherence and invasiveness of Y. ruckeri in fish cell line demonstrated that these parameters depend on the cell line used [66,80,81].
3.4. Antibiotic pressure
Antibiotics are chemical compounds that are used to kill pathogenic bacteria. Antibiotics acts on some of bacterial cell mechanisms, Such as, enzyme regulation, inhibition of enzymes involved in cell wall synthesis, nucleic acid synthesis or protein synthesis. Although the efficiency of antibiotic on pathogen bacteria depend on several factors essentially, the dose and the solubility. It was reported that biofilm production hampers antibiotic diffusion and induces an altered phenotype characterized by low metabolic activity, which results in drug tolerance; such conditions promote persistent and recurrent infections [82]. These authors revealed that antibiotic exposure in the absence of nutrients can influence the non-culturable state either by contributing to its induction or by promoting its persistence. Cell wall modifications caused by vancomycin exposure could result in increased cell wall thickness, a typical VBNC feature. Moreover, since antibiotics can act as regulators of gene expression, they can inadvertently induce cell modifications leading to the VBNC state [82].
3.5. Role of biofilm in pathogenicity
Biofilms are communities of microorganisms that are formed on solid or fluid interfaces and are designed to protect individual cells, such as bacteria, from the environment. Biofilms also contribute to the success of bacteria. Recently, interest in biofilm has grown and it has been well studied in different fields and for different purposes (technology development, industrial, human well-being, depuration, eradication). In addition, different interactions occurring in the biofilm have been studied (synergy, antagonism, competition) [83–85]. In this review, we focus on the role of biofilm in relation with pathogenicity and bacterial virulence in fish. The complexity of the bacterial tools used for cell adhesion and invasion ranges from single monomeric proteins to intricate multimeric macromolecules that perform highly sophisticated functions and can be truly considered as nano-machines [86]. In order to ensure their existence and their virulence, pathogenic bacteria coordinate the expression of their different effectors in time and for bacterial aggregation, biofilm integrity and attachment to the host tissue receptors, many pathogenic Gram-negative bacteria display long adhesive fibres, called type IV pili [41]. The virulence and pathogenicity of bacteria are often enhanced when growing as a biofilm, and, in this case, the immune responses are only directed toward those antigens on the outer surface of the biofilm because antibodies and other proteins or factors present in fish serum may fail to penetrate the biofilm [12]. Furthermore, cells within the biofilm can modulate cytokine synthesis and remain hidden from antibody and complement factor recognition, and thus from subsequent white blood cell phagocytosis [20]. Moreover, fish phagocytes are unable to effectively engulf a bacterium growing within a complex polysaccharide matrix, which may result in phagocytes releasing large amounts of pro-inflammatory enzymes and cytokines, leading to inflammation but also the destruction of nearby tissues [12,87]. In aquaculture environment it is interesting to signal that fish cells present in mucosal epithelia continuously produce mucus [13], and the continuous detachment of mucus could be considered as an important way of dispersion space. Bacterial adherence to the host may involve specific interactions between a receptor and a ligand or hydrophobic interactions of the pathogenic bacteria. This mucus can be used by some bacteria as a carbon source and could mediate the persistence of the pathogen in the environment [88]. It was recently suggested that programmed cell death may maintain the stability and homeostasis of the biofilm. Indeed, it was revealed that killing and lysis occur reproducibly in localized regions in wild-type P. aeruginosa biofilms inside microcolonies by means of a mechanism that involves a genomic prophage of P. aeruginosa. It was proposed that cell death plays an important role in subsequent biofilm differentiation and dispersal, as surviving cells may benefit from the nutrients released during bacterial lysis [89]. However, other roles for the death of a subpopulation of biofilm cells may be envisaged [90], and possible explanations for auto-inhibition have been proposed: to provide nutrients for resistant subpopulations (such as those that have reached the stationary growth phase), or to aid in dispersal and colonization of new substrates. The depletion of a substrate or accumulation of an inhibitive waste product might cause some bacteria to enter a non-growing state in which they are protected from killing [41]. Interestingly, it was recently reported that active starvation contributes to tolerance during infection, as nutrients become limited when they are sequestered by host defences, and consumed by proliferating bacteria. Starvation in biofilms is due to nutrient consumption by cells located on the periphery of biofilm clusters and by the reduced diffusion of substrates through the biofilm [91]. Biofilm bacteria show extreme tolerance to almost all antibiotic classes, and supplying limiting substrates can restore sensitivity [92]. Thus, for pathogens, autolysis (which appears undesirable for a single-cell organism) may be advantageous to a bacterial population at the multicellular level [90]. Biofilm bacteria can be up to a thousand times more resistant to antimicrobial stress than free-swimming bacteria of the same species, as previously indicated [93]. Biofilms provide bacteria with resistance to antimicrobial agents and host defences [94]. Aeromonas spp. evolved multiple regulatory mechanisms for biofilm formation that are intimately linked with the production of virulence factors. The QS response regulator of the reclassified isolate A. piscicola A1 (formerly A. hydrophila), ahyRI, produces LuxRI homologs, N-(butanoyl)-L-homoserine lactones (BHL), and N-hexanoyl-L-homoserine lactones (AHL) and autoinducers that regulate cell division [49,95]. Similarly, the recently characterized autoregulatory two-component signal transduction system, QseBC, is a widely conserved system within A. hydrophila with reduced swimming and swarming motility, which forms thicker biofilms and secretes fewer virulence factors, leading to the attenuation of virulence [49]. Baquero et al. [96] reported that resistance profiles of aquatic pseudomonads depend on the species composition, but also on the site in which they were isolated, being more antibiotic-resistant along shorelines and in sheltered bays than in the open water. Jefferson et al. [97] suggested that the biofilm partially inhibits penetration of the antibiotic, delaying it sufficiently to induce the expression of genes within the biofilm that mediate resistance. The resistance of pathogenic bacteria, forming a biofilm, to the antibiotic is explicated by the transition of the colony from exponential to slow or without growth/persisters phenomena. The Glycocalyx matrix through the efflux system and enzymes, inactivate antimicrobial agents and protect the peripheral region of the biofilm. Interestingly, cells in the intermediate position of a biofilm starve for a particular nutrient and slow their growth [98]. Besides, the antibiotic-degrading enzymes in the matrix may lead to binding and/or deactivation [99]. For pathogenic bacteria, biofilms can also exhibit resistance to host phagocytic defences. Lam et al. [100] used transmission electron micrographs of clinical specimens to show that the biofilm is surrounded, but not penetrated, by antibodies and inflammatory cells. In the same context, diffusion studies showed that IgG remained bound to the periphery of biofilm clusters and failed to penetrate the EPS matrix [101]. Another interesting interaction phenomenon that may occur in the biofilm is the transfer gene between bacteria and may be significant for the transfer of resistance genes to associated susceptible bacteria. Gene transfer can convert a previously avirulent strain into a virulent pathogen [88]. For these reasons, new and more virulent microbial phenotypes may be expressed when growing within a biofilm. Biofilms promote genetic diversity and maintain the high cell density needed for efficient genetic exchange. Several bacteria have plasmids that allow them to possess diverse characteristics. These plasmids can be transferred horizontally by conjugation to different species present in a biofilm [14,102]. Likewise, mobile genetic elements (MGEs) are a means to transfer genetic information (DNA) among and within bacterial species [103].
Importantly, MGEs encode putative virulence factors and molecules that confer resistance to antibiotics. MGEs may consist of insertion sequences, transposons, phages, plasmids, pathogenicity islands, and chromosome cassettes. These segments of DNA are largely propagated by vertical gene transfer, which is transmission of genetic information from parent to progeny cell [103]. In a study about virulence of A. hydrophila, it was demonstrated that prophages that contain putative cis-acting elements and trans-acting factors were be conserved within hypervirulent strains of A. hydrophila which strongly suggest that the differential regulation of virulence factors (and therefore a dramatic increase in virulence) may be caused by the lysogenic conversion of this conserved A. hydrophila lineage by these mobile genetic elements [104].
3.6. Other parameters
It was revealed that the magnitude of ECP expression of pathogens depends on the route of entry. The ECP of A. salmonicida produced by the cellophane overlay method reproduced the lesion normally associated with the chronic form of furunculosis (necrosis, oedematous swelling) at the site of injection in rainbow trout. However, when injected intraperitoneally, the ECP proved to be fatal for the fish [105,106]. It was reported also that the pathogenicity and adhesion was less influenced by cell line type in A. salmonicida. In fact, the S-layer also acts as an adhesion, promoting high levels of adherence to non-phagocytic fish cell lines [107]. The capsular polysaccharide is involved in the ability of A. salmonicida and A. hydrophila to adhere to and to invade fish cell lines, being an important factor for intracellular invasion [41]. It is well known that the stress is one of the parameters that influence the fish defense status and consequently increase the vulnerability of fish to pathogenic bacteria. The reaction of fish cells to a stressor agent depends on the stressor, the gene and on the organ. Effectively, when fish were stressed, the cell expression of tcr-b, csf-1r and hep genes was down-regulated in head kidney (HK). The genes gr, tcr-b and il-1b were down regulated in liver; the genes gr and il-1b were down regulated in skin; the gene cox-2 was up-regulated in skin after exposure to different stressors [108]. Another parameter influencing the pathogenicity is the intracellular lifestyles of the pathogen. The infection mode of some cells occurred through an external interaction between the pathogenic bacteria and the fish host cell. However, other pathogens including viruses, protozoa and some pathogenic bacteria such as Vibrio anguillarum, are intracellular pathogens that replicate within the cytoplasm or intracellular compartment of particular host cells. The intracellular survival of such pathogenic bacteria allowed them to escape antibodies neutralization and macrophage phagocytosis. These intracellular pathogens have mechanisms for both entering and leaving host cells [109]. Beside the parameters influencing pathogenicity cited above, many lacking information makes the mechanism of loss and recuperation of virulence for some bacteria still an ambiguous process. It was reported that electrolyzed water, with oxidant concentration of 30 ppm and pH of 2.6 showed a similar bactericidal effect as 150 ppm chlorinated water of pH 9.3, and provided a better bactericidal effect than 5 ppm ozonated water of pH 6.6 [110]. Chlorination process influences bacterial pathogenicity and it was suggested that specific mechanisms may exist for the survival of certain bacteria and viruses in waters containing relatively high concentrations of chlorine [111].
4. Interaction of pathogenic bacteria with fish cells
Disease in fish is a complex process involving the interaction of a pathogen bacterium, a susceptible fish host and environmental factors, as mentioned above. Bacterial fish infection is normally caused by opportunistic bacteria, facultative pathogen bacteria or obligate pathogen bacteria. Opportunistic bacteria invade immunosuppressed fish, while atypical fish environmental stressors, such as those occurring in intensive aquacultural rearing (hypoxia, abnormal pH, and high population density) generate an optimal setting for such pathogens to thrive. Invading fish with opportunistic bacteria has important and preventive measures to reduce host stress, along with active interventions to enhance (or restore) the protective effect of the useful microorganisms (e.g. using prebiotics, probiotics, synbiotics) [112]. Facultative pathogen bacteria replicate in an environmental reservoir such as water or soil and only cause disease if they happen to encounter a susceptible host [109]. In contrast, obligate pathogens do not require the host be immunocompromised or injured. Pathogens have developed appropriate mechanisms to avoid biochemical barriers, to access the host cell and even to use host cell mechanisms for survival and multiplication. In general, the survival, multiplication, interaction and pathogenicity of the bacteria require that the pathogen and host exist in the same environment and that the pathogen (1) successfully invades the host, (2) finds a nutritionally compatible niche in the host body, (3) has the capacity to overcome the host immune response, (4) can use the host cell mechanisms to multiply, (5) can spread to a new host and, finally, (6) possesses a resistance strategy. Obviously, the virulence and pathogenicity mechanisms developed by bacteria may be associated to the bacterial structure (membrane, fimbriae, pili,etc …), to the extracellular products and to the host cell defence capacities. Fig. 1 represents a summary of the interaction mechanism that occurs between fish host cell and pathogenic bacteria.
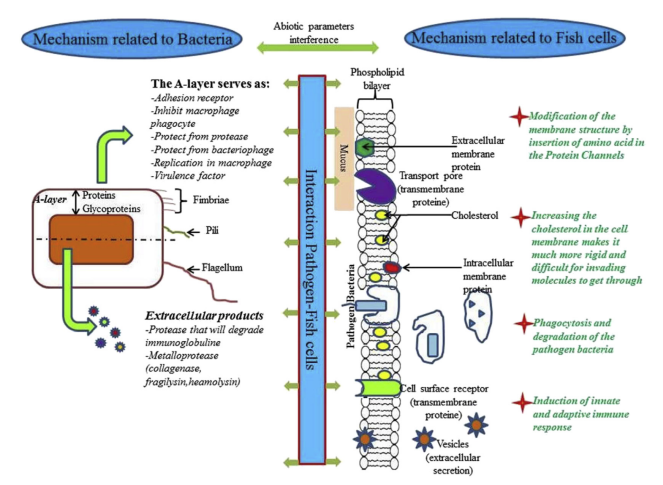
Fig. 1. The interaction between the pathogen bacteria and the host fish involves several mechanisms related to the bacteria, the host cells and other abiotic factors.
4.1. Interaction mechanisms related to the bacterial cell structure
Once existed with the fish host in the same environment, the bacteria will enter in contact involving several components such as the Alayer, the fimbriae and the pili. The A-layer (or S-layer) is an additional external layer on the cell wall. The A-layer allows the pathogen Aeromonas salmonicida to survive in the macrophage [113]. The virulence gene in A. salmonicida is called (vapA) and encodes the A-protein. Lund and Mikkelsen [114] identified a difference in the amino acid composition between typical and atypical isolates, with greater homogeneity among the former and greater heterogeneity among the latter. It was observed that virulent strains possessed an A-layer, whereas avirulent isolates did not possess an A-layer [115]. The A-layer was associated with weak or strong agglutination properties of the fish erythrocytes and, depending on the temperature; the A-layer contributes to the adhesion of A. salmonicida to cultivable cells of fish tissue [116]. Thus, adhesion is considered as the primary step in the pathogenic process. Other studies suggested that the A-layer may confer protection against phagocytosis and hence destruction by macrophages [117]. It was noted that strains of A. salmonicida maintained in the laboratory conditions for long time did not undergo auto-agglutination and had reduced virulence [118]. The A-layer was purified from virulent isolates by Kay et al. [119], who concluded that it is a surface-localized protein with a molecular weight of 49 kDa. Phipps et al. [120] demonstrated that the A-layer is hydrophobic, covers the entire cell surface, does not possess any enzymatic activity, but constitutes a macromolecular refractive protein barrier which is essential for virulence. In an experiment involving A. salmonicida was isolated from different fish species including carp, minnow, goldfish and salmonids. Analysis of the different pattern of cell protein envelopes revealed the presence of a major protein, with a molecular weight of 54 kDa, in all auto-aggregating strains. Interestingly, if the horse serum contained in the bacterial culture medium was replaced by synthetic sea salt, there was an almost complete loss of the additional cell envelope and the auto-agglutinating ability. It was suggested that the extracellular layer protects A. salmonicida cells from the action of protease, bacteriophage, and serum complement [121,122]. Munn and Trust [123] demonstrated that bacteria with A-layer were able to multiply within one of principal phagocytic organs, e.g. the spleen. Ewart et al. [124] demonstrated that A. salmonicida is capable of internalization and replication in macrophages. In addition, possible interference of the A-layer with antibacterial peptides (magainin, cecropins and defensins) was demonstrated [125]. They are usually cationic peptides that interact with the bacterial membranes, disturbing their integrity and membrane potential [126,127]. Different Gram positive or Gram negative bacteria hold components that are involved in adhesion to host-cell. Fimbriae, flagella and protein fibrils are the components more studied [128]. Different types of fimbriae could be produced by one bacterial species. FimA, for example, is a helical cylinder protein structure capped by FimH protein which recognizes mannose-containing receptors on the target eukaryotic cell [129]. Antimicrobial Peptides (AMPs) act as an essential part of the innate immune system and have been identified in virtually all forms of life. These short, positively charged peptides have a combined pore-forming and intracellular killing effect on a broad range of microorganisms including pathogen bacteria [130]. It is also know that a very common mechanism of AMP resistance in bacteria is the incorporation of positively charged molecules into their cell surface in order to reduce the possible interactions and binding of cationic AMPs due to a physical process [131]. For pathogen Gram negative bacteria, intrinsic AMP resistance has been performed by a variety of LPS modifications. The addition of 4- aminoarabinose (Ara4N) to lipid A, which creates a less negatively charged LPS and reduces binding affinity of AMPs, is regulated by the pmrCAB and pmrHFIJKLM operons in S. typhimurium [131,132]. Other AMP resistance and virulence determinants in S. typhimurium include surA, tolB, and pgm. SurA and TolB are involved in membrane stabilization while Pgm (phosphoglucomutase) is involved in LPS biosynthesis [133,134]. It was reported also that in P. aeruginosa the arnBCADTEF operon mediates the addition of Ara4N to lipid A conferring AMP resistance [135]. In Gram-positive bacteria, AMP-resistance occurs following the incorporation of positively charged molecules into cell wall teichoic acids (TA), which play an essential role in cell division morphology, adhesion, virulence and antimicrobial resistance [131,136]. Besides membrane structure modification, other effective methods for dampening the antimicrobial effects of AMPs are efflux and proteolytic degradation. In S. typhimurium AMPs are exported by the sap (sensitivity to antimicrobial peptides) efflux system and the putative ABC transporter encoded by the yejABEF, both of which are required for virulence in mice [137]. PgtE was identified as proteases that degrade AMPs in S. typhimurium [138].
4.2. Interaction mechanisms related to extracellular products
The development of new more precise techniques and methods in the last decade has made it possible to identify many extracellular products (ECP) with regard to bacterial pathogenicity and virulence. These ECPs, mostly consisting of proteases, would facilitate the propagation of bacteria by causing extensive host tissue damage, thereby degrading host proteins to provide readily-available nutrients for bacterial growth. Moreover, ECPs could also counteract the host defence system by degrading immunoglobulins and components of the complement system [88,89]. Furthermore, some bacterial extracellular metalloproteases (such as bacterial collagenase, fragilysin, pseudolysin, vibriolysin) have been identified as virulence factors of pathogenic bacteria of fish [139], as have P1, GCAT, metalloendopeptidase AsaP1, P2 metallo-gelatinase and serine caseinase [140]. He et al. [141] demonstrated that protein content in extracellular Polymeric substances (EPS) changed at high concentrations of Mg2+or Ca2+ resulting in enhancement of the adhesion of marine bacteria and biofilm formation. Bacterial phospholipases were also confirmed as virulent factors of many fish pathogenic bacteria such as Vibrio anguillarum and A. salmonicida [113,142,143]. In fact, phospholipases are divided into several subgroups depending on their specificity for hydrolysing ester bonds in different locations in the phospholipid molecule. Phospholipases A (PLAs) cleave long chain fatty acids at sn-1 (PLA1) or sn-2 (PLA2) position of phospholipids to yield lysophospholipid and free fatty acid; phospholipases C (PLCs) cleave phospholipid into diacylglycerol and a phosphate-containing head group; and phospholipases D (PLDs) cleave phospholipid into phosphatidic acid and an alcohol [144]. In their study, Morita et al. [145] determined many different aminopeptidases from fish pathogenic bacteria. For example, leucine-aminopeptidase is produced by Streptococcus and catalyses the cleavage of amino acids from the N-terminal position of peptides and proteins. Haemolysins were confirmed to be a virulence factor of many pathogenic bacteria of fish. In Aeromonas spp., Rasmussen-Ivey et al. [49] reported that the extracellular heat-labile haemolysin (AHH1) is the most abundant of several widely distributed haemolysins (AerA, AHH1, AhyA, and Asa1) in A. hydrophila, In A. media A6 (formerly A. hydrophila) Aerolysin A (aerA) and Haemolysin A (hlyA) comprise another two-component haemolytic system, In A. dhakensis SSU, the iron dependent, fur and gidA-regulated, enterotoxin Act is the most cytotoxic virulence factor of and a core gene within A. hydrophila [49]. In V. anguillarum, two haemolysin gene clusters were identified. One gene cluster, rtxACHBDE, encodes a MARTX toxin and its type I secretion system. The second haemolysin gene cluster in V. anguillarum strain M93 S m contains the haemolysin gene vah1 flanked by two putative lipase-related genes (llpA and llpB) immediately downstream, and upstream by a divergently transcribed haemolysin-like gene (plp) that appears to function as a repressor of vah1-dependent haemolytic activity [144,146]. ADP-ribosylating toxin, AexT is an ECP which was defined as an important virulence factor of many Gram-negative bacteria, including the pathogenic Yersinia spp., Salmonella spp., Shigella spp., enteropathogenic and enterohaemorrhagic Escherichia coli and Pseudomonas aeruginosa [147]. A type III secretion system has been found to be responsible for the secretion and translocation of the ADPribosylating toxin, AexT, into the cytosol of cultured fish cells [148,149]. Once within the eukaryotic cytosol, effector proteins are able to disrupt the cytoskeleton or interfere with cell signaling cascades [150,151]. Inactivation of the type III secretion system has been shown to reduce the cytoxicity of A. salmonicida subsp. salmonicida towards fish cell lines [149]. Another conserved type II secretion system (T2SS) is present in all known members of A. hydrophila and is integral in the extracellular secretion of a wide array of virulence factors including aerolysin, amylases, DNases, and proteases [49,152,153]. Indian major carp disease associated with bacterial haemorrhagic septicaemia, infectious dropsy, red mouth disease and ulcerative conditions, among others, was considered to be caused by the acetylcholinesterase produced by Aeromonads as a virulence factor [154]. In Gram negative bacteria, lipopolysaccharide (LPS) is the major component of the outer leaflet of the outer membrane (OM). LPS has three structural regions: Oantigen, core, and lipid A. Lipid A is the biologically active component of LPS recognized by the innate immune system [46]. In Aeromonads, many other ECPs were identified such as collagenase, serine protease, metalloprotease, pore-forming RTX toxin RtxA, enolase, and lipase [49]. In addition, Toranzo and Barja [142] revealed that different exoenzymes (haemolysins, cytotoxins, and dermatotoxins) can contribute to the development of infections, metalloproteases, and undetermined low molecular weight substances are the main toxins responsible for the lethality of the of ECP of V. anguillarum. Interestingly, in the same study the authors demonstrated that ECP from Renibacterium salmoninarum are devoid of proteolytic, haemolytic, and cytotoxic activities, and are not lethal for fish. The virulence of R. salmoninarum was strongly correlated with the nature and properties of the cell envelope such as hydrophobicity, auto-aggregation, haemagglutination, and the presence of a 57 Kd antigenic protein [142]. Austin and Zhang [155] revealed that ECP of V. harveyi, when heated (100 °C for 10 min) or digested with proteinase K, produced the same pathology as crude, untreated ECP. Western blotting demonstrated that all the ECP preparations contained low molecular weight lipopolysaccharide (LPS), which was considered to constitute the lethal toxin of V. harveyi.
4.3. Interaction mechanisms related to the fish cell
The intimate contact between fish and the aquatic environment make these animals exposed to the pathogenic bacteria. The mucous and the skin surfaces constitute frontline barriers limiting the invasion by pathogenic bacteria. In response to the strategies evolved by the pathogenic bacteria to adhere and get in, the host cells proceed by the development of defense mechanisms occurring at the level of these barriers. Several proteins components of the membrane of eukaryotic cell serves for cell communication, transport pore or cell receptor. Pathogenic bacteria could adhere to the eukaryotic cell receptor using one or more component cited above (membrane, fimbriae). It has been reported [156] that Staphylococcus aureus produces a specific adhesion receptor for extracellular protein and serum proteins, of eukaryotic cells, like collagen, fibrinogen and fibronectin. Several studies reported that the sugar of glycoproteins possesses an affinity to fimbria of some bacteria. In fact, mannose-containing glycoproteins, fucose and galactose act as receptor ligands for different fimbriae of E. coli to eukaryote cell [157]. Similarly, Wang and Leung [158] demonstrated that different strains of V. anguillarum differ in the types of receptors used. Two invasive strains of the organism, G/Virus/5 (3) and 811218-5 W adhered strongly to three different fish tissue culture cell lines. Adherence of strain G/Virus/5 (3), and of nine other vibrios, was inhibited by galactose-containing sugars, but adherence by strain 811218-5 W was not affected by a range of sugars tested. As no fimbriae could be detected in either strain it was concluded that non-fimbrial adhesins were involved in both cases [158,159]. The structure of eukaryotic cell membrane is composed of a lipid bilayer with integrated proteins. The inner leaflet of the lipid bilayer comprises of liquid ordered (Lo) and liquid disordered (Ld) domains. Lo microdomains, also named as lipid rafts are enriched in cholesterol, sphingomyelin and certain types of proteins, which facilitate cell signaling and nutrient uptake. This membrane structure was identified recently in common carp (Cyprinus carpio L.) tissues by Brogden et al. [160]. The same study concluded that the lipid rafts is composed essentially by triglycerides (33–62%) and cholesterol (24–32%), phosphatidylcholine and sphingomyelin. The tight interaction between these components provides the basis of their packing and rigidity, which leads to a phase separation [161]. This tight-packing organization of lipid rafts confers their resistance to solubilization by non-ionic detergents, which allows their separation and isolation from the rest of the plasma membrane using sucrose-density gradients [162]. Plowman et al. [163] reported that lipid rafts are not pre-existing domains in which proteins dynamically partition, but rather that the formation and disassembly of raft domains is a dynamic process. Based on these data revealing dynamic of the eukaryotic cell membrane process, it seems that a deep study that focuses on the interaction of pathogens with proclaimed modification of the eukaryotic cell membrane process will be interesting. The understanding of such process could clarify more adhesion of pathogen, penetration, infection process and even eventual new treatment approach. Cholesterol is another lipid molecule influences the membranes permeability and fluidity. It was suggested that the entry of pathogens, which is mediated by lipid raft components (triglycerides, cholesterol, phosphatidylcholine and sphingomyelin) increases their chance to avoid lysosomal fusion and overpower the host immune response to sustain intracellular survival [164]. Phagocytosis is a process induced by the cell when the receptors recognize any invader, in eukaryotic cells. A transformation occurred in the macrophage membrane in order to enclose, neutralize and destroy the pathogen. Importantly, some pathogens have acquired the ability to survive and replicate within macrophages after phagocytosis. Alberts et al. [109] suggested that the disability of the macrophage to destroy the pathogen was because the pathogenic organisms have acquired genes that encode proteins that interact specifically with particular molecules of the host cells. Likewise, in other cases, the ancestor of the pathogen may have acquired the gene from its host, whereas in others, random mutation may have given rise to protein motifs that are recognized by a eukaryotic protein partner. AMPs, also called cationic peptides because of their positive charge, interact with the negatively charged cell membrane of bacteria and fungi. This interaction results in pore formation and membrane perturbation, leakage of cellular components, and cell death [130]. Fish are a great source of these peptides, as they express all of the major classes of AMPs, including defensins, cathelicidins, hepcidins, histonederived peptides, and a fish-specific class of the cecropin family, called piscidins. As with other species, the fish peptides exhibit broad-spectrum antimicrobial activity, killing both fish and human pathogens [165]. The antibacterial activity of AMPs is dose-dependent and bactericidal activities are usually observed at μM concentrations. Evidence for their antibacterial efficacy in vivo comes from studies using infections in animal models [131]. AMPs are produced by epithelial cells and by circulating immune cells including neutrophils and macrophages, and are among the first immune effectors encountered by an invading microbe [166]. There are significant differences in the lipid composition of bacterial and eukaryotic cell membranes that could explicate the selectivity of AMPs for bacterial membranes, killing bacterial pathogens without damaging host tissue. In fact, bacteria have anionic lipids exposed on the surface whereas eukaryotic membranes sequester anionic lipids in the monolayer facing the interior of the cell. Although it not well elucidated yet, the general believe of the mechanism of action of AMPs is the pore formation in the bacterial cytoplasm membrane. One pore formed in the membrane, ion flows will be disturbed and membrane lipid bilayer will be altered resulting in bacterial death. Among eukaryotic cells AMPs synthesis, induction mechanism of function, human cells are more studied. The AMPs interaction with human pathogen bacteria was reviewed by Andersson et al. [131]. Schmitt et al. [167] suggested that bacterial LPS, lipoteichoic acid (LTA) and the peptidoglycan precursor lipid II represents specific components of bacterial membranes that are targeted by AMPs. AMPs activity may also involve intracellular targets after passing over the cell membrane and to block essential cellular processes without causing extensive membrane damage [168]. Several bacterial pathogens have evolved to survive and replicate within host cells after invasion. Indeed, Francisella noatunensis subsp. orientalis for example is a Gram-negative facultative intracellular bacteria that causes emergent disease in fish called Francisellosis. The range of host cell types, in which pathogens bacteria can survive, include non-phagocytic cells (such as epithelial and endothelial) and professional phagocytes (such as macrophages and neutrophils) [169]. Some pathogenic bacteria remain within a membrane-bound compartment and manipulate this subcellular site to their advantage, others have evolved to escape from the vacuole and continue their life cycle within the cytosol [170]. Independently of the mechanism of host cells invasion, once the bacteria get in the host cell, they are internalized within a membrane-bound vacuole. Under normal circumstances, the vacuole progressively acidifies as it develops into a mature degradative phagolysosome. Some pathogens survive in this niche either by preventing vacuole–lysosome fusion or by modifying the environment within the phagolysosome [170]. The professional phagocyte uses several mechanisms to kill the pathogenic bacteria such as the production of reactive oxidative intermediates, the lowering of pH of bacteria-containing vacuoles, and the activation of degradative proteases [169]. It is believed that Gram negative and Gram positive bacteria may activate a common pathway of events that lead to septic shock which is the result of the combined action of cytokines, complement components, and coagulation cascade components. Bacterial cell wall derived constituents can induce the host to produce or activate these mediators [169].
5. Therapeutic strategies for disease prevention and treatment
Aquaculture around the world has increased significantly to supply the demand for seafood, thus, the aquaculture is progress and more interest is given to related process. Fish farmer are using intensive systems that allows higher stocking densities. Nevertheless, this is generating permanently stressful conditions that threaten fish welfare and significant challenge rises to fish farming is disease caused by pathogenic bacteria. Given that bacteria can survive in the environment without need of hosts, bacterial diseases is easy to encounter in aquaculture. For prophylactic and therapeutic treatments farmers apply drug supplemented feeds to keep fish free of diseases. however, the indiscriminate uses of antimicrobial agents, has led to the development of the resistant strains and the need to switch over the other antibiotics [6]. Diseases caused by antibiotic-resistant increases resistance of bacteria to antibiotic and an additional challenge for fish immune system is rising. Other threats for fish are found in the environment such as the pesticides, heavy metals and other pollutants could influence fish health and predispose them to infections. Therefore, a combined approach that considers not only the pathogen but also the host and the environment will be the most effective method to improve fish health.
- Disinfection of the tanks and all equipment that will be used before the introduction of the fish.
- Larvae should be bought healthy from a certified source and quarantined in isolated tanks.
- Disinfection of the fish larvae with an antiseptic products (e.g. Potassium permanganate), with salt bath for fish of fresh water to treat injured fish and/or prevent them from parasites localized in the skin surface or in the gills.
- It is well recommended to use separate tanks to decrease the impact of potential disease outbreak.
- Fish vaccination is strongly advisable to stimulate and enhance immune system performance against potential pathogen infections.
- Preventive maintenance of all the equipment of culture system (e.g. pumps, filters)
- Constant control of the water quality and environmental parameters (eg. Temperature, oxygen). - Uses of turbine or other mechanisms to prevent biofilm formation.
- Prevent all stress factors during fish management.
- Keep out of the group all fish presenting minor clinical signs and prevent introduction of disease to healthy fish.
- Fish should be fed with a complete nutrient supplemented with vitamins and minerals.
- Fish food must be stored in hygienic conditions to prevent pollution, contamination, deterioration of the quality.
- Adopt a general strategy of hygiene and surveillance.
6. Conclusions
Several pathogenic bacteria have been isolated from outbreaks affecting fish species. While pathogens bacteria in fish farms are currently controlled by the administration of antimicrobial agents. However, the excessive use of antimicrobials has led to the emergence of antibiotic resistant bacteria. This review underlines the diversity of the mechanisms controlling pathogenicity from adhesion, parameters influencing virulence to interaction with host cells. We focus on the bacterial adhesion which is a crucial step in host colonization and infection, in biofilm formation. Pathogen bacteria use several components from their surface structures to achieve adhesion. The controls of these components are governed by a cluster of genes that modulate their synthesis, the cell-cell and cell-host interaction, the biofilm formation, development and architecture. Although the diversity of virulence mechanisms that depends on the pathogenic species, the advances in molecular biology and sequencing of complete genome improved the understanding of virulence process and the strategies used by pathogens to escape host neutralization. Advanced studies in virulence mechanism confirm its reliance to biotic and abiotic factors such as bacterial modulation to viable but nonculturable (VBNC) state, temperature, role of biofilm, antibiotic pressure, nutrient availability and other environmental stress factors. In order to defend themselves from infectious diseases, fish possesses various immunological strategies including humoral and cellular pathways, modulation of membranes permeability and fluidity, production of antimicrobial peptides. A deeper knowledge of the interactions occurring between the host fish and pathogen bacteria (and parameters influencing the virulence mechanisms) will provide new knowledge for the refinement of existing treatment strategies to combat biofilm infections and for the development of novel treatment strategies.