Induction of Regulatory Responses by the Microbiota
Maintenance of tissue homeostasis is imperative for host survival. This fundamental process relies on a complex and coordinated set of innate and adaptive responses that selects and calibrates responses against self, food, commensals, and pathogens in the most appropriate manner. To this end, specialized populations of cells have to integrate local cues such as defined metabolites, cytokines, or hormones, allowing the induction of responses in a way that preserves the physiological and functional requirements of each tissue.
As such, the regulatory pathways that are involved in the maintenance of a homeostatic relationship with the microbiota are likely to be tissue specific. However, most of our current understanding of commensaldependent regulatory pathways relates to the gastrointestinal environment. In the gut, the formidable challenge represented by the exposure to the microbiota, food-derived antigens, metabolites, and pathogens requires a highly complex network of regulatory pathways that is only beginning to be understood (Figure 2). Failure to regulate these responses can lead to severe pathological outcomes ranging from inflammatory bowel diseases (IBD) to allergies or, as further discussed, metabolic syndromes.
Commensals are a critical and active inducer of regulatory responses. Notably, the establishment of tolerance—the active suppression of inflammatory responses to food and other orally ingested antigens—could not be induced in the absence of gut flora-derived signals (Kiyono et al., 1982; Sudo et al., 1997; Wannemuehler et al., 1982; Weiner et al., 2011). Although immunological tolerance is likely to be achieved via multiple and redundant mechanisms (Weiner et al., 2011), over the past few years, Foxp3+ regulatory T (Treg) cells have taken central stage in our understanding of this process. These cells maintain both peripheral and mucosal homeostasis throughout the lifespan of the host, and disruption of the homeostasis of these cells results in loss of oral tolerance and development of aberrant effector responses in the gut (Josefowicz et al., 2012a; Mucida et al., 2005; Worbs et al., 2006).
Although Foxp3+ Treg can arise as differentiated cells in the thymus, the gastrointestinal tract environment is a privileged site for the induction of Treg cells in response to oral antigens (Coombes et al., 2007; Mucida et al., 2005; Sun et al., 2007). A current consensus is that optimal maintenance of tolerance to commensal and environmental antigens requires the combined effect of both thymically and GI-induced Treg (Cebula et al., 2013; Josefowicz et al., 2012a; Lathrop et al., 2011). This specialized property of the gut to induce Treg can be, at least in part, explained by the presence of specialized populations of antigen-presenting cells, such as the CD103+ CD11b+ DC. Notably, these gut-resident dendritic cells are endowed with the capacity to produce factors involved in the induction of Treg, such as the cytokine TGF-b and the vitamin A metabolite retinoic acid (RA) (Coombes et al., 2007; Mucida et al., 2007; Sun et al., 2007).
Tissue-specific factors such as vitamin A and MUC2, a mucus glycoprotein produced by intestinal goblet cells, contribute to the regulatory specialization of mucosal dendritic cells (Klebanoff et al., 2013; Shan et al., 2013). The importance of this pathway for the control of mucosal homeostasis is highlighted by the finding that a proportion of induced Treg in the colonic tissue is specific for antigens derived from the commensal microbiota (Lathrop et al., 2011). Induction of Treg cells is proposed as one of the mechanisms of action of probiotics—defined bacteria known to confer a health benefit to the host. Indeed, some of the regulatory effects of probiotics in the context of inflammatory diseases and atopic eczema in neonates and infants is believed to be associated with the induction or expansion of Treg (Di Giacinto et al., 2005; Feleszko et al., 2007; Karimi et al., 2009; Zoumpopoulou et al., 2008) and the manipulation of mucosal DCs toward a proregulatory function (Foligne et al., 2007; Smits et al., 2005).
Commensals can also control oral antigen sampling by mucosal DCs and promote the induction of lamina propriaresident macrophages associated with local expansion of Treg cells (Chieppa et al., 2006; Niess and Adler, 2010). Aside from the direct influence of the microbiota on the immune machinery associated with the induction of oral tolerance, commensalspecific Treg can promote class switching to IgA in an antigenspecific manner (Cong et al., 2009; Tsuji et al., 2009), thereby controlling the host relationship with the microbiota via multiple mechanisms (Peterson et al., 2007; Suzuki et al., 2004) (Figure 2). The first demonstration that a unique symbiont molecule could promote regulatory responses was provided by the identification of the polysaccharide A (PSA), which is produced by a prominent human symbiont Bacteroides fragilis (Mazmanian et al., 2005).
B. fragilis, via PSA expression, can protect mice from experimental colitis induced by Helicobacter hepaticus, a commensal bacterium with pathogenic potential (Mazmanian et al., 2008). This protective activity was associated with the capacity of PSA to induce and expand IL-10-producing Treg cells (Mazmanian et al., 2008; O’Mahony et al., 2008; Ochoa-Repa´raz et al., 2010a). B. fragilis was able to promote Treg cell function and induction via engagement of the microbial-derived PSA with TLR2 expressed by T cells, a phenomenon associated with the capacity of this bacterium to also limit Th17 responses (Round et al., 2011).
The discovery of a link between defined members of the microbiota and the induction of regulatory cells able to limit mucosal inflammation and promote tolerance led to a rational approach for the identification of the next generation of probiotics with superior capacity to induce Treg cells. Induction of Treg cells is not restricted to B. fragilis, as the presence of an indigenous Clostridium species also promotes Treg cell accumulation via, at least in part, its capacity to create a TGF-b rich environment (Atarashi et al., 2011).
Of note, optimal induction of Treg in the colonic environment relies on the synergistic effect of a consortium of Clostridia strains, whereas individual species have a modest effect on the immune system (Atarashi et al., 2013). Based on the fundamental role of Treg in maintaining mucosal homeostasis, it is likely that, rather than being restricted to defined commensals, a large fraction of any given microbiota or microbiota metabolism may have evolved to favor this aspect of the regulatory network. Indeed, recent findings have shown that many organisms can increase the frequency of Tregs in the colon (Faith et al., 2014; Geuking et al., 2011).
Mammals rely on bacteria to break down undigestible dietary components such as fiber (Ley et al., 2006a). One dominant metabolite resulting from this process is short-chain fatty acids (SCFA), ubiquitous bacterial fermentation products that are highly enriched in the colonic environment (Cummings et al., 1987). Although a role for SCFA in controlling various aspects of immune responses has been long recognized (Meijer et al., 2010), their link to lymphocyte function has only recently been appreciated. Notably, SCFA and, in particular, butyrate regulate the size and function of the regulatory T cell network by promoting the induction and fitness of regulatory T cells in the colonic environment (Arpaia et al., 2013; Furusawa et al., 2013; Smith et al., 2013). Butyrate is well known to regulate gene expression epigenetically by inhibiting histone deacetylases (HDACs) (Davie, 2003), and this property is currently proposed as an underlying mechanism for enhanced Treg generation in the gut.
The action of SCFA likely results from the manipulation of various cells involved in the induction of regulatory responses, and indeed, the effect of SCFA on both T cell and dendritic cells has been linked to this process (Arpaia et al., 2013; Furusawa et al., 2013; Smith et al., 2013). Altogether, these results reveal a major role for the microbiota in shaping the repertoire, number, and activation of tissue-resident Treg cells and in the maintenance of host-microbe mutualism at barrier sites. Based on the abundance and complexity of the flora, one could also speculate that opportunity for cross reactivity between commensals, pathogenic, and environmenta antigens is high.
Thus, microbial pressure in the gut could lead to the induction and maintenance of a pool of activated Treg cells that may not only contribute to the maintenance of a mutualistic relationship with the microbiota, but also to the systemic control of immune responses. One of the first demonstrations of the protective role of commensals during acute injury was provided by the observation that, in the gut, TLR activation by commensals was required to promote tissue repair and host survival (Rakoff-Nahoum et al., 2004). Part of the protective effect of the microbiota in the context of inflammation relates to its capacity to sustain the aforementioned regulatory network (Arpaia et al., 2013; Bouladoux et al., 2012; Furusawa et al., 2013; Smith et al., 2013). Commensal-derived products can also act by controlling directly or indirectly the function of inflammatory cells. For instance, recognition of the commensal-derived metabolites SCFA by innate immune cells is critical for the regulation of inflammation in response not only to intestinal injury, but also in models of arthritis and allergy (Maslowski et al., 2009). Furthermore, commensals can also tune the function of inflammatory monocytes, a population of cells involved in the control of pathogens (Figure 2). During acute mucosal infection, encounter of inflammatory monocytes with the microbiota in the gastrointestinal tract promotes their production of the lipid mediator PGE2 that, in turn, limits the level of activation of tissue-damaging neutrophils (Grainger et al., 2013). Although most of what is known today about the regulatory properties of the microbiota arises from the exploration of the bacterial component of the flora, other microbes such as fungi and virus are likely to promote similar or complementary aspects of the regularly network. In the gastrointestinal tract, interaction of commensal fungi with the C-type lectin receptor Dectin 1 was able to prevent inflammation in the context of acute mucosal injury (Iliev et al., 2012).
Induction of Protective Responses by Commensals
Tissues that are natural habitats of the microbiota such as the skin, the GI tract, or the lung are also the portals by which pathogens access the host and, often, the primary site of infections. This implies that the initial encounter of pathogens with the immune system occurs in an environment conditioned and regulated by its endogenous microbiota. As such, the fate of commensals and pathogens (as well as their classification) is highly interdependent (Figure 3).
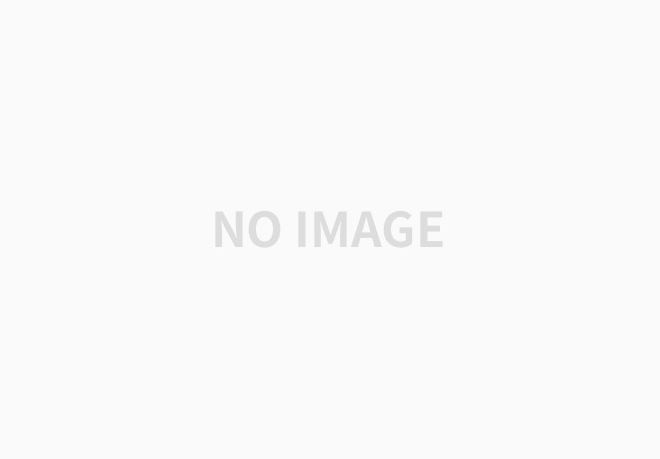
Figure 3. Promotion of Protective Immunity by the Microbiota The symbiosis between the microbiota and its mammalian host encompasses multiple relationships, including mutualistic, parasitic, and commensal. The capacity of a given microbe, including those composing the microbiota, to trigger or promote disease is highly contextual, and some microbes can shift from mutualist to commensal to parasite according to the state of activation of the host, co-infection, or localization. Commensals can control microbes with pathogenic potential (as normal constituent of the microbiota or acquired) via distinct mechanisms. Commensals can compete for nutrients and produce antimicrobial molecules and metabolites that affect the survival and virulence of pathogens. Commensals can promote the production of antimicrobial peptides by epithelial cells and reinforce tight junctions. Finally, commensals can modulate the function of dendritic cells and other innate cells both locally and systemically in a manner that promotes the induction of effector T and B cells responses against pathogens. When uncontrolled, this adjuvant property of the microbiota can promote inflammatory and autoimmune disorders.
Notably, commensals can directly and dynamically interact with pathogens and immune cells, and the results of this interaction can define the pathogenesis and outcome of a given infection. Protection of the host from exogenous pathogens by commensal bacteria, a phenomenon referred to as colonization resistance, was described more than five decades ago (Buffie and Pamer, 2013; van der Waaij et al., 1971).
One of the major forms of interaction between the microbiota and invading microbes relates to the need for both forms of organisms to compete for the same ecological niche. Consequently, commensals have been shown to limit pathogen colonization through competition for defined metabolites in a process referred to as colonization resistance (Kamada et al., 2013). Alteration of nutrient availability by the host microbiota can also have profound consequences on the expression of virulence genes and growth rate of pathogens such as enterohemorragic E. coli or Clostridium difficile (Kamada et al., 2013; Ng et al., 2013; Pacheco et al., 2012).
Manipulation of microbial virulence can also occur as a consequence of commensal metabolism. In some instances, the very same metabolites involved in the manipulation of the immune system, such as SCFA, can also directly act on pathogens by downregulating the expression of virulence genes such as those encoding the type 3 secretion system in Salmonella enterica and typhimurium (Gantois et al., 2006). Commensals can also promote the establishment of an environment hostile for pathogen establishment, with the best illustration in the vaginal environment in which lactobacilli can protect from pathogenic colonization via reduction of the local pH (Turovskiy et al., 2011). Finally, commensals can also produce antimicrobial peptides that directly affect pathogen growth or survival (Hammami et al., 2013).
For instance, E. coli produces bacteriocins—proteinaceous toxins that specifically inhibit the growth of the same or similar bacterial strains—thus impairing the growth of the related pathogen, enterohemorrhagic E. coli. Additionally, the dominant skin commensal Staphylococcus epidermidis produces several antimicrobial proteins and proteases that can limit the biofilm formation of Staphylococcus aureus (Iwase et al., 2010; Schamberger and Diez-Gonzalez, 2002) (Figure 3). In tandem with its direct action on invasive microbes, the microbiota’s capacity to control infection is also associated with its remarkable ability to promote and calibrate both innate and adaptive immunity (Molloy et al., 2012). Indeed, commensals play a fundamental role in both the training of the immune system and its functional tuning, thereby acting as adjuvants to the immune system as a whole.
As previously discussed, commensals can reinforce defined components of barrier immunity, an effect that has the dual function of promoting their own containment and limiting pathogen invasion. For example, the commensaldependent antimicrobial peptide RegIIIg not only contributes to maintenance of segregation between the microbiota and host epithelial cells, but also promotes protection against defined pathogens, such as vancomycin-resistant Enterococcus faecalis (Brandl et al., 2008; Vaishnava et al., 2008, 2011). An important role of the microbiota is associated with its capacity to condition cells to respond to infectious challenge both systemically and locally. For instance, commensals can tune innate cells in a way that allows them to rapidly respond to pathogen encounters. An example of this control is provided by the capacity of the gut microbiota to control the production of IL-1b, a cytokine that is involved in host defense. The microbiota contributes to the homeostatic production of pro-IL-1b by intestinal resident macrophages in a MyD88-dependent manner, thereby priming these cells to respond rapidly to enteric infections by conversion of pro-IL-1b to mature active IL1b (Franchi et al., 2012).
Some of the stimulatory effects of the microbiota can be attributed to dominant microbial-derived signals. Indeed, commensals and pathogenic microbes interact with the host immune system through conserved ligands that are immutable features of microorganisms (Sansonetti and Di Santo, 2007). Many of these ligands signal through the Toll-like and Nod-like families of receptors (Sansonetti and Di Santo, 2007; Takeda et al., 2003). One example of this is flagellin, a structural protein that forms the main portion of flagella and promotes bacterial chemotaxis, adhesion, and invasion of host tissue by pathogenic bacteria. Flagellin is also expressed by a large number of commensal bacteria, and several lines of evidence indicate that interaction of commensal flagellin with Toll-like receptor 5, in particular in the context of barrier breach, plays an important role in the promotion of mucosal immunity. Notably, dendritic cells that reside in the lamina propria of the intestine are poised to respond to flagellin by rapidly expressing chemokines and antimicrobial peptides, as well as cytokines involved in the initiation of immune responses (Kinnebrew et al., 2012; Uematsu et al., 2008; Uematsu et al., 2006).
In response to flagellin, CD103+ DC produce IL-23, in part, to induce IL-22 by innate lymphoid cells (ILCs), thereby promoting epithelial-mediated host defense (Kinnebrew et al., 2012). Unmethylated cytosine phosphate guanosine (CpG) dinucleotides, which are abundant within the prokaryotic DNA of intestinal flora, can contribute to intestinal homeostasis under steady-state conditions (Hall et al., 2008), and constitutive interaction between CpG-expressing commensal DNA and TLR9 can act as a local adjuvant of immune responses (Hall et al., 2008).
Nonetheless, under homeostatic conditions, both inflammatory and regulatory signals are constantly integrated—the sum of these signals leading to the establishment of an immune tone compatible with tissue immunity. The capacity of the microbiota to stimulate innate responses translates into its important role in the induction of adaptive immunity (Figure 3). Indeed, early studies have identified significantly impaired host immune responses to pathogens in mice treated with antibiotics or raised under germ-free conditions (Cebra, 1999; Hall et al., 2008; Ivanov et al., 2009; Mazmanian et al., 2005). One of the first demonstrations of this ‘‘adjuvant’’ effect was revealed in a parasitic model of small intestine infection with Encephalitozoon cuniculi in which protective Th1 and Th17 responses were severely compromised in the absence of commensals (Hall et al., 2008).
Similarly, protective Th17 responses failed to develop in response to Citrobacter rodentium, a model of attaching and effacing infection that primarily affects the large intestine (Ivanov et al., 2009). The adjuvant property of the microbiota has also been revealed in a model of oral vaccination (Hall et al., 2008), an observation that may help to explain some of the failures of oral vaccination in developing countries in which malnutrition and infection have profoundly affected the microbiota (Korpe and Petri, 2012). In addition to the pleiotropic effects of conserved microbial ligands or metabolites in the education and function of the immune system, it is now becoming clear that unique microbes or groups of bacteria can dominantly influence immune system development and function under steady-state and inflammatory conditions. In the language of ecological systems, these organisms of paramount importance are termed ‘‘keystone species.’’
The prototype of a keystone species in the GI tract is represented by the interaction between segmented filamentous bacteria (SFB) and the immune system. Under steady-state conditions, this spore-forming Gram-positive anaerobic bacteria colonizes the terminal ileum of mice. SFB has a dominant effect on the mucosal immune system by promoting the accumulation of both Th17 and Th1 cells in the small intestine and driving the production of IgA (Gaboriau-Routhiau et al., 2009; Ivanov et al., 2009; Umesaki et al., 1999).
In contrast to most commensals that reside outside of the ‘‘demilitarized zone,’’ SFB interacts closely with the mucosal tissue via tight adhesion to Peyer’s patches and epithelial cells, inducing cytoskeletal reorganization in these cells at the site of contact (Ivanov et al., 2008; Sczesnak et al., 2011). This intimate contact with epithelial cells, a property shared by a select minority of commensal organisms, is believed to account for the capacity of SFB to heighten tissue immunity and promote protective responses to pathogens (Ivanov et al., 2009). The presence of bacteria with a keystone role on the immune system raises an intriguing possibility. Although optimal control of host metabolism and physiology may rely on complex and redundant populations of microbes, we could speculate that a more limited number of microbes may act as adjuvants of immune responses. Indeed, overpopulation of the GI tract with bacteria that have enhanced inflammatory potential can have local and systemic pathological consequences.
Under steadystate conditions, these ambivalent members of the microbiota, such as E. coli or SFB, are maintained in check by the immune system and coopted by the host to control invasive microbes. This protective effect of the microbiota has been revealed in clinical and experimental settings in which broad antibiotic treatment can allow the domination of intestinal microbiota with drug-resistant microbes such as vancomycin-resistant enterococcus (VRE), a pathogen that causes bloodstream infections in immunocompromised patients (Buffie and Pamer, 2013; Murray, 2000). Infections caused by multidrug-resistant organisms are on the rise and have developed into endemic and epidemic situations worldwide (Gupta et al., 2011). Harnessing the microbiota to combat these infections represents an important therapeutic avenue, with the most spectacular results obtained thus far in the context of Clostridium difficile colitis (van Nood et al., 2013). During this recurrent infection, transfer of a microbiota from a healthy donor eradicated the infection with a remarkable efficiency (van Nood et al., 2013). Again highlighting the concept of defined microbes endowed with superior adjuvant capacity, the protective effect of the microbiota in VRE-infected patients was highly dependent on the presence of the commensal Barnesiella (Ubeda et al., 2013). Thus, the microbiota is a required component of the effector response of the host. Although currents studies are attempting to link defined microbes to unique immunological states in human, the microbiota is a highly dynamic and complex composite of microbes all expressing a large number of potential ligand and metabolites. Under homeostatic conditions, both inflammatory and regulatory signals are constantly integrated—the sum of these signals leading to the establishment of a controlled inflammation compatible with tissue immunity. Therefore, under most settings, changes in inflammatory state associated with the microbiota are unlikely due to a single microbial product or metabolite but may result from a shift in the balance of signals.
Systemic Control of Protective Immunity
Though it is readily accepted that shifts in the gut microbiota composition and density can affect local immune responses, it is becoming apparent that these changes can also alter immunity and inflammation in organs distal from the intestine (Belkaid and Naik, 2013). For instance, reduction of gut commensals via broad-spectrum antibiotic treatment results in blunted T and B cell response against intranasal infection with influenza (Ichinohe et al., 2011). This effect of the microbiota can be linked to its capacity to promote the inflammasome-mediated induction of IL-1b and IL-18 secretion (Ichinohe et al., 2011). In this setting, rectal administration of TLR agonists restored protective immune responses, indicating that either the microbial products are capable of diffusing systemically or that inflammasome activation does not need to occur at the site of infection (Ichinohe et al., 2011). Antibiotic treatment also impaired adaptive and innate antiviral responses following exposure to systemic lymphocytic choriomeningitis virus (LCMV) and musosal influenza virus (Abt et al., 2012). Genome-wide transcriptional profiling of macrophages from antibiotic-treated mice revealed a broad decrease of genes associated with antiviral immunity (Abt et al., 2012). This bystander control of peripheral responses can be, at least in part, explained by the unique requirement of the GI tract for absorption resulting in the constant diffusion of low-level microbial products such as TLR or NOD ligands and metabolites into the bloodstream. For instance, commensalderived peptidoglycan can be found in the serum and has been shown to improve the killing of Streptococcus pneumonia and Staphylococcus aureus by bone-marrow-derived neutrophils in a NOD1-dependent manner (Clarke et al., 2010). Experimental evidence suggests that the tonic sensing of commensal products or metabolites present in the blood stream also contributes to steady-state hematopoiesis and monocyte egress from the bone marrow (Maslowski et al., 2009; Shi et al., 2011). Recent evidence implies that the capacity of commensals to calibrate systemic immunity has profound consequences in the context of immunotherapy. Total body irradiation, used in defined settings of immunotherapy and bone marrow transplantation, is associated with gut damage and microbial translocation, providing an adjuvant effect to the transferred anti-tumoral T cells (Paulos et al., 2007). A similar mechanism is proposed to explain the protective role of the microbiota in the context of chemotherapy. Cyclophosphamide, a clinically important cancer drug, also leads to intestinal damage, bacterial dysbiosis, and translocation and induction of anti-commensal Th17 responses that collectively contribute to the anti-tumoral response (Viaud et al., 2013). Some of the effect of the microbiota can also occur independently of gut damage. Indeed, disruption of the gut flora via antibiotic treatment or in germ-free mice impairs the capacity of the host to control subcutaneous tumors during immunotherapy (Iida et al., 2013). In these experimental settings, the protective effect results from the capacity of the commensalderived ligands to control the status of activation of tumor myeloid cells and, more particularly, their level of TNF-a and reactive oxygen species, both associated with optimal tumor control (Iida et al., 2013). Remarkably, tumor control was associated with the presence of defined commensal species such as Alistipes shahii (Iida et al., 2013). Thus, commensals and more particularly defined member of the microbiota can control various aspects of immunity associated with anti-tumoral responses, an effect that has profound clinical implications. Altogether, these data reveal that exposure to microbial ligands shapes systemic immunity at both the steady state and in the context of inflammation. The basal tuning of the immune system associated with constant sensing of microbial products or ligands implies that subtle changes in this conditioning may have long-term consequences on the capacity of the host to mount systemic immune responses and develop inflammatory diseases. The mechanism underlying this phenomenon remains incompletely understood, but we could speculate that commensal bacteria-derived signals can influence gene expression profiles of immune cells via epigenetic modifications of genes involved in innate responses, thus creating a transcriptional state that enables basal expression of host-defense factors and rapid responses upon encounter with a pathogen. How permanent the effects of microbial sensing are and to what extent tissue and hematopoetic stem cells are permanently influenced by this tonic sensing remain to be explored.
Compartmentalized Control of Tissue Immunity
Although the above mentioned observations propose that gut commensals can control the systemic threshold of activation of innate and adaptive cells, these studies do not exclude a direct role for commensals residing in the lung, skin, or other barrier sites in the control of local immunity. Indeed, microbial surveys unveiled the remarkable partitioning of commensals within the human body, with each tissue and microenvironment hosting unique microbial communities (Belkaid and Naik, 2013). Thus, each barrier tissue is a complex and in some cases unstable composite of microbes and host structural, hormonal, nervous, and immunological networks, with each of these systems potentially controlled by resident microbiota. The skin, the largest organ of the body, represents a critical interface between the host and the environment. Unbiased microbial sequencing has shown the presence of highly diverse and specific commensal niches along distinct topographical sites of the skin (Costello et al., 2009; Grice et al., 2009). Although the skin is a rather inhospitable environment—poor in nutrients and moisture—up to 1 billion bacteria inhabit a typical square centimeter of human skin, covering the surface and extending down into the sebaceous glands and hair follicles (Grice et al., 2008). In contrast to the known role of the gut microbiota in promoting the gastrointestinal-associated lymphoid tissue (GALT) development, skin commensals are not required for the development of associated lymphoid tissue (Naik et al., 2012). The skin-resident bacteria, such as Staphylococcus epidermidis, can control fundamental aspects of local immunity and tissue repair (Lai et al., 2009; Naik et al., 2012). Skin commensals do not affect the capacity of T cells to be primed or to migrate to the skin but modulate dermal T cell function by tuning the cutaneous inflammatory milieu and, more particularly, the production of IL-1a that, in turn, directly controls the capacity of dermal resident T cells to produce inflammatory cytokines such as IFN-g and IL-17A (Naik et al., 2012). Thus, in contrast to the role of the gut microbiota, the action of skin commensals on the local immune system is discrete and highly compartmentalized. The oral cavity also harbors a unique and complex microbial community accumulating on both the hard and soft oral tissues in sessile biofilms (Avila et al., 2009). One of the proposed roles of the oral microbiome on the immune system is associated with its capacity to promote inflammasome activity, leading to the local increase of the inflammatory cytokine IL1b (Dixon et al., 2004). At other sites, such as the lung or vaginal mucosa, the role of commensals on tissue immunity remains largely unknown. In the absence of commensals, the number of infiltrating Th2 lymphocytes and eosinophils was elevated, and the composition and status of activation of lung dendritic cells were altered during airway inflammation (Herbst et al., 2011). Additionally, intranasal priming of mice with live or heat-inactivated Lactobacillus can dampen local responses, thereby protecting against lethal sequelae infection with the virulent pathogen pneumonia virus of mice (PVM) (Gabryszewski et al., 2011; Garcia-Crespo et al., 2012). These results are consistent with the notion that commensal bacteria, in most tissues, can establish a threshold of activation and regulation required for immune fitness. Nonetheless and despite the growing number of studies that associate commensal dysbiosis at diverse tissue sites with specific pathologies such as psoriasis, atopic dermatitis, and asthma (Abreu et al., 2012; Belda-Ferre et al., 2012; Hilty et al., 2010; Kong et al., 2012; Srinivasan et al., 2010), little is known about the effect of these unique microbial communities in the control of tissue-specific immunity. Based on our understanding of tissue specialization, we could postulate that these unique microbial communities have coevolved with their host to finely tune the unique environment of each tissue site.
Role of the Microbiota in the Pathogenesis of Infection: The Accidental Pathogen
As previously discussed, pathogenicity of most microbes is a contextual state. Owing to the adjuvant capacity of the microbiota, infections that occur at sites colonized by commensals can be, in some cases, considered as co-infections, with normal constituents of the microbiota being a major culprit of tissue damage and pathogen transmission. Although tissue-resident symbionts can provide an immunological and ecological shield against pathogen invasion, these microbes can in some instances be coopted by pathogens for optimal transmission. One of the first illustrations of the positive effect of the flora on pathogen development and survival was revealed in a model of Trichuris muris nematode infection (Hayes et al., 2010). In this model of infection, egg hatching in the large intestine only occurred upon tight contact with bacteria, suggesting that the microflora provide critical cues for the appropriate establishment of the life cycle of gut tropic nematodes (Hayes et al., 2010). This pro-infection role of the microbiota represents a novel paradigm for the transmission of various pathogens, including viruses. Poliovirus relies on the microbiota for efficient replication, an effect that can be at least in part associated with the capacity of the virus to bind to cardinal microbial products such as LPS (Kuss et al., 2011). Similarly, the capacity of MMTV to bind to commensally derived LPS favors mucosal transmission of the virus via the induction of the regulatory cytokine IL-10. Such effects lead to a state of immunological unresponsiveness toward viral antigen that favors transmission of the virus (Kane et al., 2011). In these studies, the elements accounting for the pathogen transmission and/or virulence-promoting effect of the flora are highly represented microbial products such as LPS, suggesting that both virus and nematodes may have evolved to bypass commensal population shifts by thriving on ubiquitous microbial derived components. This would suggest that, although manipulating the flora may represent an efficient way of altering immunity to pathogens, this strategy is unlikely to have global consequences on pathogen transmission. Based on the pleitropic effect of the flora in induction of regulatory pathways, host metabolism, and tissue-resident function, one would expect that a high number of pathogens transmitted via mucosa or using commensal-rich habitats have evolved to benefit from the complex interaction of the host with its microbiota. Infections represent highly volatile situations for barrier tissues, with pathogens, commensals, and environmental antigens transiently sharing the same inflamed environment. In westernized countries, it is estimated that a child will suffer ten diarrheal episodes on average before the age of 5, a number that can be dramatically increased in the developing world (Kosek et al., 2003; Vernacchio et al., 2006). Thus, when added together with skin and lung infections, the immune system has ample opportunity to be exposed to commensals during inflammation, a situation that has the potential to disrupt our homeostatic relationship with these microbes. In the gastrointestinal tract, acute mucosal infections are characterized by dysbiosis associated with significant shifts in the microbiota and dominance of bacteria, with enhanced invasive and inflammatory properties that can directly exacerbate inflammation and tissue damage (Egan et al., 2012; Heimesaat et al., 2006; Lupp et al., 2007; Stecher et al., 2007). In particular, g-proteobacteria dominance has emerged as a hallmark of acute mucosal infections and enhanced pathology (Benson et al., 2009; Craven et al., 2012; Egan et al., 2012; Heimesaat et al., 2006; Lupp et al., 2007; Molloy et al., 2013; Raetz et al., 2013; Stecher et al., 2007). Various mechanisms could contribute to proteobacterial ‘‘blooms,’’ including the capacity of these commensals to thrive on metabolites derived from inflammatory setting such as nitrates and benefit from the selective death of Paneth cells (Raetz et al., 2013; Winter et al., 2013). Because of the pathogenic role of these bacteria, the immune system may have evolved specific modes of control. Indeed, in the context of acute mucosal infection, both neutrophils and monocytes can exit the lamina propria and enter the gut lumen, thereby creating a containment structure referred to as intraluminal cast, which limits epithelial contact with these invasive microbes and translocation (Molloy et al., 2013). In a number of models of gastrointestinal infections, such as Toxoplasma gondii and Yersinia pseudotuberculosis, immunopathology can also induce the translocation of commensal bacteria (Brenchley and Douek, 2012; Brenchley et al., 2006; Estes et al., 2010; Hand et al., 2012; Heimesaat et al., 2006; Jung et al., 2012; Meinzer et al., 2012). A consequence of infection that results from the induction of inflammation and enhanced microbial translocation is that, in contrast to steady-state responses, commensal-specific T cells, much like pathogen-specific cells, become activated and differentiate to an inflammatory phenotype (Hand et al., 2012). In the GI tract, these commensalspecific T cells form memory cells that are phenotypically and functionally indistinguishable from pathogen-specific T cells (Hand et al., 2012). Because of the extraordinary number of potential antigens expressed by the host microbiota, this implies that a significant fraction of memory cells are expected to be commensal specific and will develop over time in response to successive infections and/or various barrier breaches. Thus, primary exposure to a pathogen in the skin, lung, and GI tract is likely to occur in the context of a much broader recall response against commensal bacteria. Much like pathogen-specific CD4 T cells, commensal-specific memory T cells declined steadily over time (Hand et al., 2012; Homann et al., 2001; Pepper et al., 2010). As CD4 T cells carry out the complex task of remembering and responding to pathogenic and commensal organisms in a constantly changing environment, perhaps development of CD4 memory reflects this necessity for flexibility. An evolving pool of specificities within the regulatory and effector CD4 T cell compartment may allow for the maintenance of tolerance and barrier function in the face of variable commensal populations and intermittent infection. The physiological consequence of long-term T cell memory against commensals remains to be addressed. One possible consequence may be the induction of heterologous memory, wherein antigen-specific responses against commensal bacteria could drive the rapid production of inflammatory cytokines upon secondary infection, leading to increased protection against both pathogens and commensal bacteria. In support of this hypothesis, healthy human serum contains antibodies that are specific to the skin and intestinal microbiota (Haas et al., 2011). In addition, recent studies suggest that CD4 T cell clones that are cross-reactive to commensals and viruses are common in healthy individuals (Su et al., 2013). Conversely, aberrant accumulation of commensal-specific T cells may lead to the development of inflammation and IBD (Sartor, 2006). A further exploration of antigen-specific memory T cells residing at all barrier sites and their regulation would inform us of the potential of impact of effector responses against commensals at steady state or in the context of inflammation.
Role of the Microbiota in Chronic Diseases
Optimal microbiota host interaction implies that balance between stimulatory and regulatory signals would allow the development of immunity without compromising the capacity of the host to maintain tolerance to innocuous antigens. Yet, in westernized countries, the overuse of antibiotics, changes in the diet, and elimination of chronic parasitic infections, such as those caused by helminths, including roundworms, hookworms, and whipworms, may have selected for a microbiota that lacks the resilience required for the establishment of balanced immune responses. For instance, as recently as 1940, the prevalence of helminthic worm infection in children in some rural areas of the United States was as high as 70% (Johnston et al., 2014; Weinstock et al., 2004). Further, the use of antibiotics and changes in diet have caused the chronic disappearance of potentially critical components of the human microbiota (Blaser and Falkow, 2009). These profound changes in the microbiota and, as a direct result, the immune system are now believed to contribute to the dramatic and rapid increase in chronic inflammatory and autoimmune disorders seen in high-income countries. Indeed, though each inflammatory disease is associated with unique genetic and biological mechanisms, a unifying trend seems to be that many inflammatory diseases are associated with significant shifts in the resident microbiota from a ‘‘healthy’’ to ‘‘diseased’’ state. One tantalizing hypothesis is that the partially penetrant nature of genetic polymorphisms associated with many complex diseases is due to the necessity of changes in the microbiota for the onset of pathology. An alarming consequence of this hypothesis is that susceptibility to some diseases is partly due to the stochastic acquisition of specific commensal organisms. Interestingly, similar ‘‘opportunists’’ are associated with multiple disease states. Therefore, some bacteria may be particularly adept at surviving in and contributing to inflammation, and the recent acquisition of a particular set of inflammatory clades of bacteria may be an important contributor to the etiology of inflammatory and autoimmune diseases. Below, we will discuss a number of diseases and their associations with the microbiota.
'Role of the Microbiota in Immunity and' 카테고리의 다른 글
Role of the Microbiota in Immunity and Inflammation (0) | 2024.12.23 |
---|