Transepithelial transport mechanisms
Transfer of substances across the intestinal epithelium can occur through either the transcellular route or the paracellular route. Paracellular transport is thought to be due to diffusion and solvent drag. The solvent drag hypothesis has been proposed by Pappenheimer (1993), and predicts that sugars and amino acids may, in part, be transported paracellularly.
The proposed mechanism consists of transcellular sodium ion uptake across the epithelium which creates high osmolality in the lateral spaces. Water would thus be transferred through the TJs, which in turn could bring hydrophilic nutrients across the TJ through bulk flow (Pappenheimer 2001). The solvent drag hypothesis has been criticized by many as being of little if any physiological importance (Ferraris and Diamond 1997; Kellett 2001) and will thus not be discussed further in the present thesis.
Paracellular diffusion of substances is driven by electrochemical gradients. The diffusional rate is limited by the TJ permeability, which can differ between tissues. The anterior region of the salmonid intestines has higher diffusion rate than the posterior region (Schep et al. 1998).
Water transport
Intestinal water transport in the vertebrates is thought to be driven by osmotic gradients only, as no active water transporters have been found in any organism. There is still some controversy, however, about which route water crosses the intestinal epithelium and how this is regulated (Loo et al. 2002; Reuss and Hirst 2002).
The “standing-gradient” hypothesis (Figure 3C) predicts water transport to occur across the epithelium by the force of local osmotic gradients which are actively created in the lateral spaces of the epithelium. According to this view, solutes (mainly sodium), is actively secreted from the enterocytes into the lateral space between adjacent enterocytes to create a high local osmolality. This local hyperosmolality draws water from the lumen transcellularly and through the TJ to varying degree (Diamond 1979; Holtug et al. 1996). Models of intestinal water transport conflicting with the standing-gradient hypothesis have emerged (Reuss and Hirst 2002).
The major recent controversy is regarding the role of “water pumps” as some authors call the sodium-coupled glucose carrier SLGT1 (Loo et al. 2002). Intestinal SLGT1 transporters have been suggested to transport 200-260 water molecules together with the single glucose molecule and the two sodium ions, resulting in a stoichiometry of 70- 90 molecules of water for each solute. This uptake can take place against an osmotic gradient because it is driven by the electrical and chemical gradient for sodium, thus earning the name “water pump” (Schultz 2001). This mechanism has been suggested to account for half of the intestinal water uptake in humans (Loo et al. 2002). The water pump hypothesis have been criticized, and the stoichiometric coupling is claimed to be explained entirely by osmotic flow (Duquette et al. 2001; Schultz 2001). Further research is needed to confirm or discard the water pump hypothesis. Aquaporines (AQP) are water channels which greatly increase the cell membrane permeability for water in several tissues. Currently, at least six aquaporine isoforms have been found in the digestive system and four of these (AQP3, 4, 8 and 9) are expressed in the intestinal epithelium of mammals (Matsuzaki et al. 2004). The hypothesis has been proposed that aquaporins in the intestinal epithelium could be the primary route for water absorption within the standing-gradient hypothesis (Schultz 2001). There is, however, little experimental proof supporting the view that AQP are important components of epithelial water uptake in the intestine of mammals (Matsuzaki et al. 2004). In fish, reports are emerging on the presence of aquaporins in the intestine (Santos et al. 2004). The Japanese eel (Anguilla japonica) expresses at least two aquaporine isoforms in the intestinal epithelium which are similar to the mammalian AQP1 (Aoki et al. 2003) whereas the European eel (Anguilla anguilla) expresses AQP1 and AQP3 homologues in the GI tract (Lignot et al. 2002; Martinez et al. 2005b). The function of eel AQP have been suggested to include epithelial water uptake, and that the AQP is regulated for the purpose of euryhaline osmoregulation (Aoki et al. 2003).
Nutrient uptake
Nutrients can cross the membrane of epithelial cells by diffusion or by membrane transporters (Figure 3B). The diffusion rate increases linearly with concentration, whereas transport by membrane transporters becomes saturated at higher concentrations. Energy for nutrient uptake against an electrochemical gradient can be harnessed from ions (usually Na+) moving with an electrochemical gradient. The actual source for the energy is the ATP hydrolysis by the Na+,K+-ATPase (Collie 1995) removing intracellular Na+ and thus creating the electrochemical Na+-gradient (Ferraris and Diamond 1997).
In fish, a few membrane-bound nutrient transporters have been found. Glucose is transported by a transporter protein with similar functional and genetic characteristics as the mammalian glucose transport system (SGLT1 in the BBM and GLUT2 in the basolateral membrane); (Buddington et al. 1997; Collie 1995). In carnivorous fish, such as the salmonids, the rate of sugar absorption is low with little scope for up-regulation (Buddington et al. 1997; Clements and Raubenheimer 2006). Amino acids (AA) can be transported as free AA’s, as small peptides, or as larger proteins. Mammalian AA transporters are Na+-dependent or Na+- independent. The four categories of AA’s, neutral, basic, acidic and imino, are transported by the corresponding Na+-dependent BBM transporter (Silk et al. 1985; Thomson et al. 2001a). In addition, there are two Na+-independent transporters, one for neutral and basic AA’s and another one for acidic AAs (Ray et al. 2002). The presence of Na+-dependent AA transporters in fish has been confirmed (Figure 3B), although the studies are few and scattered over several species of fish, making general conclusions difficult (Collie 1995). Di- and tripeptides have been suggested to constitute a large part in the absorption of digested protein, and Na+-independent pathway has been suggested (Buddington et al. 1997; Collie 1995). Protein endocytosis occurs in the posterior intestine of several fish species (as described below) (Ezeasor and Stokoe 1981; Georgopoulou et al. 1988) which may serve nutritional purpose (Vernier 1990) as well as immunological (as discussed below). Lipophilic substances can cross the epithelial cells by diffusion through the lipid bi-layers. Most of the lipid uptake in fish occurs in the pyloric caeca and anterior intestine, whereas the posterior intestine is suggested to have less lipid absorptive function (Vernier 1990). The major dietary lipids are triglycerides, whereas phospholipids and cholesterol amount to only a few percent (Olsen 1997). Digested triglycerides and phospholipids are absorbed into the enterocytes (Figure 3B) as free fatty acids (FFA), glycerol and 2-monoglycerides (2-MAG). In mammals, transport of FFAs across the BBM has been suggested to be partly mediated by transmembrane fatty acid transport proteins (FATP) (Stahl 2004; Thomson et al. 2001a). After entry, FFA and 2-MAG are converted into complex lipids, mainly triacylglycerol (TAG) and phospholipids (PL), before being packaged in lipoproteins and exported into the circulation of the lymphatic system. Long-chain fatty acids (LCFA), mainly polyunsaturated fatty acids (PUFA) can be transferred to the circulation as FFAs (Thomson et al. 1993). In fish, the lipid uptake is thought to occur in a similar manner (Olsen 1997; Oxley et al. 2006). Macromolecule and particle transport across the mammalian intestinal epithelium can occur through pinocytosis and phagocytosis (Figure 3A), with subsequent exocytosis on the opposite side of the epithelium (Kucharzik et al. 2000). In fish, phagocytotic epithelial cells have been found (Hart et al. 1988; Ringø 2006; Ringø et al. 2003; Vernier 1990). Phagocytosis of bacteria, occurs in all regions of the salmonid intestinal tract including the pyloric caeca (Ringø 2006; Ringø et al. 2001; Ringø et al. 2003). However, the posterior intestine has been suggested to have higher phagocytotic activity than the other intestinal regions (Clements and Raubenheimer 2006; Hart et al. 1988; Olsen et al. 2001; Rombout 1998).
The pathogen Aeromonas salmonicida
Furunculosis
Furunculosis is a bacterial disease in salmonids. Fish can acquire either chronic or acute furunculosis. Acute furunculosis is characterized by septicaemia (blood poisoning) without external symptoms, usually followed by death; whereas the chronic form includes characteristic furuncle boils as well as darkening of the skin, lethargy, anemia and paling of the gills. Internally, there can be inflammation of the intestinal blood vessels, paling of the liver, swelling of the spleen, and the kidney can become liquefied. The fish loose apetite, leading to empty intestines (Inglis et al. 1993). Aeromonas salmonicida is the gram-negative bacteria causing both the acute and the chronic forms of furunculosis. Five A. salmonicida sub-species have been isolated; salmonicida, achromogenes, smithia, masoucida and pectinolytica (Cipriano and Bullock 2001; Pavan et al. 2000). The present thesis focuses on the subspecies A. salmonicida subsp. salmonicida which is also termed typical A. salmonicida and which cause typical furunculosis.
Virulence factors
A number of bacterial factors are essential for virulence by the A. salmonicida. These factors can be divided into exotoxins, endotoxins, adhesion proteins and type III secretion systems (Figure 4).
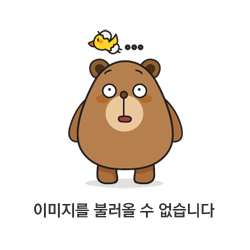
Figure 4. Structures of the A. salmonicida envelope. The insert figure shows shape and size of A. salmonicida. A part of the bacterial wall is enlarged to present the surface and associated structures. Lipopolysaccharide (LPS) and S-layer proteins covers the external surface. Type II secretion systems, Type III secretion system, Type IV Pili and porins are also present on the surface.
The exotoxins and endotoxins are extracellular products (ECP) found in the bacterial medium. The cell envelope of A. salmonicida is covered by the S-layer, a outer surface layer of proteins (Garduno et al. 1995). The S-layer confers virulence (Beveridge et al. 1997; Noonan and Trust 1997) by the proposed mechanisms of improving adhesion to the host cell membranes (Garduno et al. 2000) and by providing resistance to protease digestion (Noonan and Trust 1997).
Present on the surface are also long extruding type IV pili (Figure 4), known from studies on other Aeromonas species in mammals to be an adhesion factor to intestinal epithelial cells and possibly also to other bacterial cells (Kirov et al. 1999). Similar pili have been found in A. salmonicida and also demonstrated to be important virulence factors for infection in the rainbow trout (Masada et al. 2002). The possible importance of type IV pili in epithelial adhesion and epithelial translocation in fish has not yet been elucidated. A large variety of exotoxins, i.e. toxins actively secreted from the bacteria into the surrounding medium, have been described for A. salmonicida (Ellis 1991; Gudmundsdottir et al. 2003). One group of toxins consists of membrane damaging toxins (lysins) including the glycerophospholipid: cholesterol acyltransferase (GCAT) (Bricknell et al. 1997; Gudmundsdottir et al. 2003; Lee and Ellis 1990). The GCAT forms large (2000 kDa) complexes with lipopolysaccharide (LPS). These complexes have been considered to be among the most potent of the examined extracellular products. The GCAT:LPS complex lyses several cell types, but mortality is correlated with the hemolytic activity, indicating that anemia is one of the major causes of death (Lee and Ellis 1990). Another group of A. salmonicida toxins are the proteases, including a collagen digesting metalloprotease (Arnesen et al. 1995), and the serine protease P1 (also called caseinase) which is the major proteinaceous component of the ECP (Ellis 1991). Functionally, the serine protease may digests proteins for bacterial nutrient uptake, but it may also cause thrombosis (Arnesen et al. 1995; Ellis 1991) and immune system suppression (Hussain et al. 2000) in the host animal. However, genetic deletion of GCAT and serine protease did not reduce virulence after IP injection. Nor did the deletion reduce infectivity as assessed by cohabitant challenge (Vipond et al. 1998), which suggests that the major exotoxins are not involved in the primary entry of the host. Endotoxin or lipopolysaccharide (LPS) is another major component of the ECP (Ellis 1991). LPS may not have enzymatic functions or direct toxicity (Schletter et al. 1995), but is a highly potent inducer of the immune system. The resulting inflammation can be harmful and even fatal in mammals (Munford 2005). Recently, a TTSS has been found in A. salmonicida. Functionally, the TTSS of A. salmonicida is relatively unknown. Burr (2005) has demonstrated that knocking out the TTSS gene expression of A. salmonicida completely abolished virulence after injection in rainbow trout, compared to injections of wild-type A. salmonicida. One virulence mechanism shown to be lost in the TTSS knockout mutant A. salmonicida is the ability to avoid phagocytosis by leukocytes, but it is possible that other TTSS-dependent virulence mechanisms are also lost (Burr et al. 2005). It has not been tested if A. salmonicida use TTSSs for crossing the epithelial barrier in salmonids.
Bacterial Translocation - How and Why
The question of the major infection route for A. salmonicida in salmonids has been addressed by researchers using several different techniques, but the subject has not been satisfactory settled. Experimental infection have shown that disruption of the integument by artificial wounding can increase the risk for systemic infections and mortality in Atlantic salmon (Svendsen and Bøgwald 1997). Other studies suggest that the gills may be an infection route (Ellis 1997; Inglis et al. 1993). Several studies have isolated A. salmonicida from the intestinal lumen of salmonids (Cipriano et al. 1997; Hiney et al. 1994; Markwardt and Klontz 1989), suggesting that the pathogen can reach and colonize the intestine. Infections through the oral route have been demonstrated (Cipriano and Bullock 2001; Rose et al. 1989), whereas other studies have reported resistance against orally induced infections (Perez et al. 1996; Tatner et al. 1984). With the bacteria capable of colonizing the intestinal tract, the question then arises of whether it can cross the intestinal barrier and infect the host. The in vitro studies performed with live A. salmonicida in the present thesis show that translocation across the barrier do occur in the isolated metabolically active and viable intestine (Papers II, IV and V).
Why does translocation occur?
Why do bacteria cross the multiple defense barriers in the salmonid intestinal wall? This issue can be discussed from two different perspectives: the bacterial and the host. For the bacteria, the evolutionary benefit for increased infectivity is the possibility to multiply to large numbers, in order to maximize spreading to new hosts, by using host nutrients. For the host, the need for specific immune defenses against pathogens may require selective sampling of gut microbes, as is known in mammals (Acheson and Luccioli 2004; Kucharzik et al. 2000). However, the antigen sampling function is at the same time used by some pathogenic bacteria to cross the epithelium. In order to reach the systemic circulation and cause infection, the bacteria must survive the host mucosal immune system which normally neutralizes translocated bacteria (Cossart and Sansonetti 2004). Avoiding macrophage phagocytosis is something that A. salmonicida has been shown capable of (Burr et al. 2005).
A proposed model of A. salmonicida translocation
A. salmonicida virulence factors (Figure 4) include several functions that may be required for passing the intestinal barriers. Virulence by A. salmonicida after IP injection at least partly require type IV pili (Masada et al. 2002). This is the same type of pili that promote adhesion to intestinal epithelial cells by other Aeromonas species, as shown in mammals (Kirov et al. 1999). It is thus possible that the type IV pili are used by A. salmonicida for attachment to the epithelial BBM (Figure 5.1), a process thought to be important for virulence among intestinal pathogenic bacteria in mammals (Berg 1995). There are high concentrations of commensal bacteria attached to the epithelial cells, both to the BBM microvilli tips and between microvilli, in salmonids (Ringø et al. 2001; Ringø et al. 2003), indicating that bacteria are able to attach to the BBM. If A. salmonicida are able to multiply and form BBM-attached colonies, secreted exotoxins could subsequently cause damage of the epithelium. Electron micrographs of epithelium exposed to A. salmonicida for 90 minutes in vitro indicate that enterocytes of the anterior region of the Atlantic salmon intestine can become detached from the epithelial sheet (Paper III). Gaps in the epithelium caused by the shedding of enterocytes could thus be a route through the intestinal epithelium (Figure 5.3). Such damage to the epithelium should, if widespread, be possible to detect as increased permeability using TER and/or permeability markers. However, extensive epithelial damage caused by the mammalian pathogen Salmonella typhimurium has not been detected as decreased TER in rat intestinal segments mounted in Ussing chambers (Kurkchubasche et al. 1998), indicating that the resistance can be upheld during bacteria-induced epithelial damage. In the present studies exposing the epithelium to A. salmonicida (Papers III, IV and V), the permeability did not noticeably increase. This suggests that the damaging effect is local. Permeability-increasing damage may also be masked by a general decrease in permeability by secretion of mucus, a process used by the salmonid epithelium as a response to A. salmonicida (Lødemel et al. 2001), and shown to cause a decreased permeability as measured by electrical resistance in salmonids (Schep et al. 1997; Schep et al. 1998). The damage to the intestinal epithelium (Paper III), has been suggested to be caused by extracellular products. The extracellular proteases may be able to affect the cell-to-cell adhesive junctions. Shedding of enterocytes may also be caused by apoptosis or necrosis after bacterial entry into the epithelial cells (Mayhew et al. 1999), as is seen in Salmonella-infected intestinal epithelia (Guiney 2005). The lack of effect on the paracellular permeability, TER and Papp after ECP exposure (Paper V) may indicate that the damage to the epithelium is caused by the bacterial cells (Paper III) instead of the suggested extracellular toxins. It is also possible that the histological damages observed are isolated events that are too rare to affect the total paracellular permeability. Extracellular products from A. salmonicida was found to reduce the transepithelial potential and the short-circuit current of intestinal segments (Paper V), indicating that extracellular products affect the ion transporting function of the epithelium. The effect could be inhibition of ion channels or pumps, or a general decrease in the energy metabolism of the cells. It is concluded that none of the major enzymes known to be secreted from A. salmonicida (described above) caused the effect, as removal of molecules larger than 10 kDa by filtration, failed to remove the effect. Unknown smaller substances are thus suggested to cause the observed decrease in active transporting mechanisms of the enterocytes. Whether the effect is harmful to the epithelium, and constitutes a bacterial strategy for affecting the epithelial barrier, is unknown. It is possible that the ECP can the energy metabolism of the cell that may lead to necrosis and thus possibly the shedding of cells (Paper III; Mayhew et al. 1999).
If disruption of the epithelium is a mechanism for bacterial translocation of A. salmonicida, it is probably not the only mechanism, as transcytosis of A. salmonicida across the intestinal epithelium is also a highly likely route (Figure 5.2). Phagocytotic entry into the epithelial cells by S. typhimurium and the subsequent disruption of the rat epithelium has been shown (Kurkchubasche et al. 1998), indicating that the two pathways are not mutually exclusive. Numerous data (Papers II, IV and V) show translocation rates independent of the P app and TER i.e. the regional pattern is different and the responses to factors can differ between these parameters. For example, the posterior intestinal region has twice the TER and half the Papp of the anterior region, whereas the translocation rate for bacteria was similar or higher in the posterior region. If paracellular diffusion (through TJ or damaged epithelia) was the main pathway for translocation, the anterior intestine would be expected to have higher translocation rates than the posterior region. The posterior intestinal epithelium of salmonids has been suggested to have higher phagocytotic and transcytotic activity than the anterior region (Hart et al. 1988; Rombout 1998). This indicates that the relatively high bacterial translocation rate seen in the posterior region is governed through transcytosis. Several bacterial pathogens in mammals use TTSS for the passage of the intestinal epithelium. After the discovery of a functional TTSS in A. salmonicida, it has been speculated that the TTSS is used during the epithelial translocation in salmonids (Paper V). Heat-inactivation (42ºC) of A. salmonicida caused a reduced ability to translocate. TTSS requires the bacteria to detect and respond to host cell contact, indicating that the secretion is actively controlled by the bacteria. As TTSS require active control over secretion (Hueck 1998), it may not function after heat-inactivation. One or several of the many proteins needed for TTSS function may thus become dysfunctional. It is also possible that some other virulence mechanisms used for translocation become damaged by the heat-inactivation, disabling the efficient translocation seen for the live bacteria. It is thus possible that TTSS is required for the initial passing of the host epithelium. The subsequent stage of surviving the host mucosal immune system is likely to be TTSS-dependent, as inhibition of macrophage phagocytosis of A. salmonicida requires TTSS (Burr et al. 2005). In mammals, LPS can have adverse effects on the epithelium. The LPS detecting function in vertebrates has evolved to produce early and rapid immunological response to infection by gram-negative bacteria. One effect of serosal LPS exposure of the mammalian epithelium is increased paracellular permeability as well as increased translocation of macromolecules and bacteria (Dickinson et al. 1999; Osman et al. 1998). In rainbow trout, serosal LPS administration in vitro caused an increased transepithelial transport of bacterial- sized plastic microspheres (Paper V). LPS lacks direct toxicity, indicating that the effect is mediated through the host immune system (Figure 5.5). Indeed, LPS from A. salmonicida has been shown to induce systemic cytokine release in mice (Gudmundsdottir and Gudmundsdottir 2001), and in rainbow trout, IP injection of E. coli LPS increased TNF-alpha mRNA expression in the intestinal mucosa after 12 hours (Niklasson 2006). TNF-alpha have been shown to increase TJ permeability in the intestinal epithelium in mammals (Schmitz et al. 1999). These observations together may suggest that the observed increase in particle transport could be mediated through a local immune system response such as proinflammatory cytokine release also in the trout intestine. The evolutionary rationale for increasing transport of bacteria and particles after LPS detection may be considered counterintuitive, but it is suggested to involve increased antigen sampling for induction of the adaptive immune system. It has also been suggested that the effect is a negative consequence of increased oxygen consumption and/or decreased blood flow in mucosal tissues, causing ischemia and acidosis in the epithelium (Haglund 1994; Salzman et al. 1994) or a consequence of loosening of TJ to promote transepithelial migration of neutrophils (Parkos et al. 1994; Reaves et al. 2001). Endothelial TJ dilate during inflammation to promote immune cell migration into damaged tissue (Tharp 1989). As the epithelial TJ respond to the same dilating signals, such as proinflammatory cytokines, the effect on epithelia may be a side-effect from the mechanism aimed at increasing the endothelial permeability. The mechanism for this epithelial increase in paracellular permeability of the mammalian intestine has been suggested to be internalization of TJ proteins into the epithelial cells as a response to proinflammatory cytokines, possibly trough pinocytosis (Chiba et al. 2006). These mechanisms have not been investigated in fish, but the increase in particle transport without effects on the paracellular transport (Paper V) indicates increased transcellular transport without changes of the TJ during the short in vitro experiments, although in a longer time perspective the permeability could be affected. If LPS is involved in the intestinal translocation of A. salmonicida, its role is likely limited to the LPS released after the initial penetration of the epithelium, as only serosal LPS-exposure causes increased transepithelial transport. LPS could thus increase the translocation of more bacteria after the initial bacteria reach the lamina propria (Thomson et al. 2001b). When reaching the lamina propria, exotoxin enzymes can digest connective tissue and exotoxin lysins lyses cells. Passage across endothelia into the host circulation may thus be aided by secreted exotoxins. Survival of A. salmonicida despite the immune system at all stages of infection is likely dependent on TTSS-mediated secretion of anti-phagocytotic proteins (Burr et al. 2005).
In conclusion, A. salmonicida expresses several of the known virulence factors utilized for transepithelial translocation by mammalian gram-negative pathogenic bacteria. In addition, translocation of viable bacteria has been demonstrated in the present thesis. The intestinal tract is thus suggested to be a functional entry point for A. salmonicida in salmonids.
'The Intestinal Epithelium of Salmonids' 카테고리의 다른 글
The Intestinal Epithelium of Salmonids - The intestinal epithelium (0) | 2024.12.01 |
---|